Uni-traveling-carrier photodiodes
Ieee account.
- Change Username/Password
- Update Address

Purchase Details
- Payment Options
- Order History
- View Purchased Documents
Profile Information
- Communications Preferences
- Profession and Education
- Technical Interests
- US & Canada: +1 800 678 4333
- Worldwide: +1 732 981 0060
- Contact & Support
- About IEEE Xplore
- Accessibility
- Terms of Use
- Nondiscrimination Policy
- Privacy & Opting Out of Cookies
A not-for-profit organization, IEEE is the world's largest technical professional organization dedicated to advancing technology for the benefit of humanity. © Copyright 2024 IEEE - All rights reserved. Use of this web site signifies your agreement to the terms and conditions.
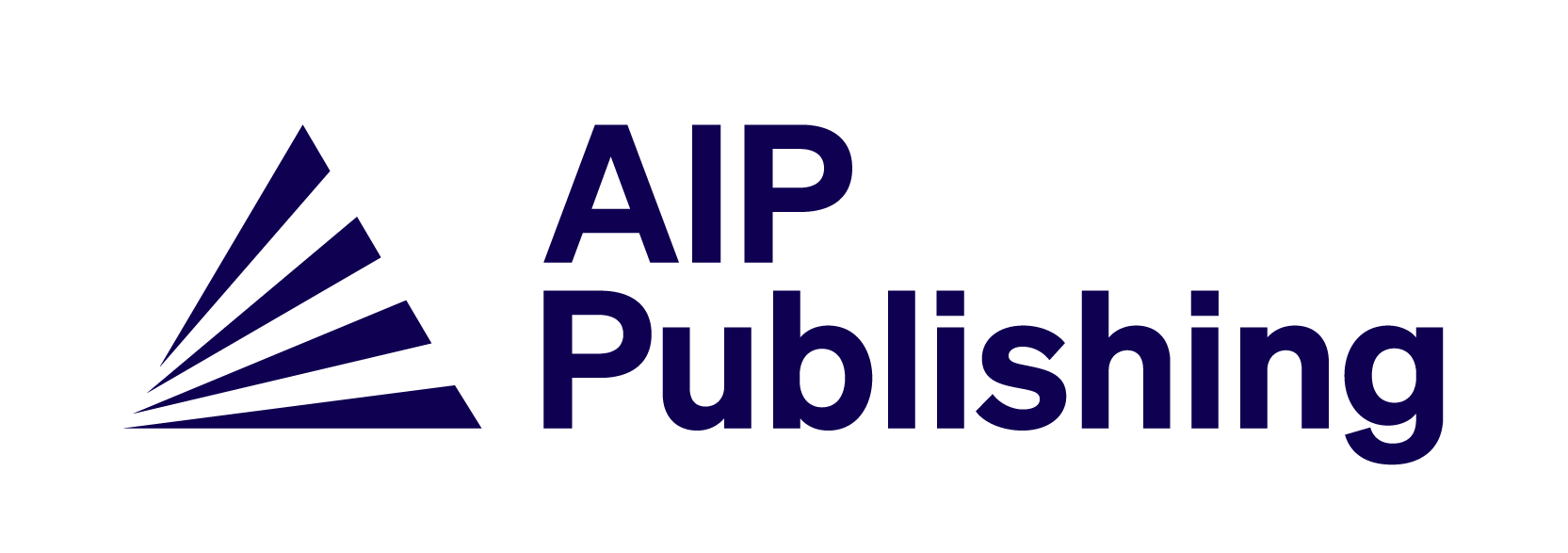
- Previous Article
- Next Article
I. INTRODUCTION
Ii. design and fabrication, iii. results, a. performance, b. terahertz communication experiment, iv. conclusion, author declarations, conflict of interest, author contributions, data availability, high-speed uni-traveling-carrier photodiodes on silicon nitride.

- Split-Screen
- Article contents
- Figures & tables
- Supplementary Data
- Peer Review
- Open the PDF for in another window
- Reprints and Permissions
- Cite Icon Cite
- Search Site
Dennis Maes , Sam Lemey , Gunther Roelkens , Mohammed Zaknoune , Vanessa Avramovic , Etienne Okada , Pascal Szriftgiser , Emilien Peytavit , Guillaume Ducournau , Bart Kuyken; High-speed uni-traveling-carrier photodiodes on silicon nitride. APL Photonics 1 January 2023; 8 (1): 016104. https://doi.org/10.1063/5.0119244
Download citation file:
- Ris (Zotero)
- Reference Manager
Integrated photonics is an emerging technology for many existing and future telecommunication and data communication applications. One platform of particular interest is silicon nitride (SiN), thanks to—among others—its very low-loss waveguides. However, it lacks active devices, such as lasers, amplifiers, and photodiodes. For this, hybrid or heterogeneous integration is needed. Here, we bring high-speed uni-traveling-carrier photodiodes to a low-loss SiN-platform by means of micro-transfer-printing. This versatile technology for heterogeneous integration not only allows very dense and material-efficient III–V integration but also eases the fabrication, yielding high-performance detectors. The waveguide-coupled photodiodes feature a responsivity of 0.3 A/W at 1550 nm, a dark current of 10 nA, and a bandwidth of 155 GHz at a low bias. At zero bias, a record bandwidth of 135 GHz is achieved. We further demonstrate that this integrated detector can be used for direct photomixing at terahertz frequencies. A back-to-back communication link with a carrier frequency of around 300 GHz is set up, and data rates up to 160 Gbit/s with a low error vector magnitude are shown, showcasing a near-identical performance at zero bias.
Integrated photonic solutions are key to solve many challenges for next-generation telecommunication, LIDAR, and quantum computing systems. More specifically, silicon nitride (SiN) platforms prevail over other materials, thanks to the very low-loss waveguides and some of the best integrated filters. 1 Unfortunately, active devices are not natively available. In recent years, the heterogeneous integration of amplifiers and lasers has been demonstrated. 2,3 However, for communication—be it telecom, datacom, or microwave photonics—the compact integration of high-speed detectors is even more important.
The widespread adoption of SiN platforms is held back by the absence of such high-bandwidth photodiodes. Table I shows a comparison of the state-of-the-art waveguide-coupled photodetectors at 1550 nm for different material platforms. Germanium on silicon 4 (Ge-on-Si) currently offers some of the best waveguide-coupled photodiodes for photonic integration, although this solution is not transferable to low-loss SiN platforms. A Ge-on-Si photodiode coupled to a SiN waveguide is demonstrated at O-band 8 but uses plasma-enhanced chemical vapor deposition (PECVD) of SiN. For true low-loss waveguides at C-band, low pressure chemical vapor deposition (LPCVD) of SiN is required. Monolithic III–V photodiodes 5,6 ——often uni-traveling-carrier photodiodes (UTC PDs)—also show very high bandwidths, but these lossy waveguide platforms are not suitable for large scale photonic integration. On the other hand, the current solutions to bring III–V photodiodes to SiN show only limited bandwidths. So far, wafer bonding has held the highest record with a bandwidth of 20 GHz. 7 Although wafer bonding can potentially reach an identical performance, it lacks the versatility of micro-transfer-printing that allows native source-wafer processing, heterogeneous integration of multiple material sources, and Back-End-Of-Line (BEOL) integration on non-flat surfaces (e.g., a local recess in the top cladding).
State of the art of waveguide-coupled photodetectors at 1550 nm.
For photocurrents above 1 mA this drops below 67 GHz.
We demonstrate a UTC PD heterogeneously integrated on a SiN photonics platform with a bandwidth of over 100 GHz, even at zero bias. This high-bandwidth bias-free operation additionally paves the way for future mmWave and terahertz wireless technologies such as on-chip remote antenna units (RAUs) that are not possible with existing platforms. For a phased antenna array to support grating-lobe-free beam steering, strict requirements are imposed on the spacing in between antenna elements and, thus, on the space available for photodiode integration. The bias-free operation eliminates the need for a DC-feed network that often cannot be sufficiently miniaturized.
To showcase the performance of our heterogeneous integration approach, we demonstrate a communication link at 300 GHz with data rates up to 160 Gbit/s. These are, to the best of our knowledge, the first 100 Gbit/s signals and beyond using bias-free optical-to-THz converters.
The UTC PDs are integrated on SiN waveguides by means of micro transfer-printing 9 ( Fig. 1 ). First, pickable chiplets—coupons—are made on a III–V source wafer and then transfer-printed to the SiN target chip, as demonstrated earlier. 9
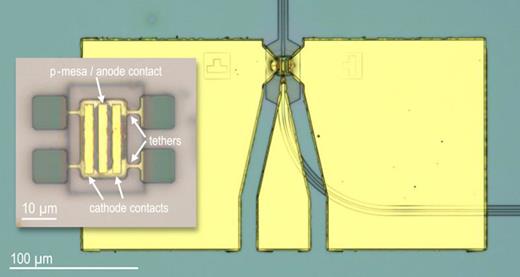
A uni-traveling-carrier photodiode (UTC PD) is integrated on a SiN platform by transfer-printing a coupon (inset) on a waveguide.
The design of the epitaxial layer stack is based on the work of Latzel et al. 10 and adapted to the micro-transfer-printing process (see Table II ). This boils down to including a 500 nm release layer between the InP substrate and the functional layers. To reduce the dishing effect of an under-etch, In 0.52 Al 0.48 As is chosen as the material for this sacrificial release layer. 11 Compared to InGaAs, it is etched isotropically and, thus, faster. This ensures that the bottom surface of the coupon is flat. The UTC epitaxial layer stack features an undoped collector of 100 nm and a 150 nm-thick absorption region with a graded composition. This results in a graded bandgap that improves the electron transport in the absorption layer by boosting the built-in electric field.
UTC epitaxial layer stack.
Graded composition.
The design of the individual waveguide photodiodes consists of a central mesa, the active layer of the photodiode that contains the absorbing and collecting layer, as well as the anode contact on top. This mesa is flanked by two cathode contacts, separated only 500 nm apart, to allow the wider base of the mesa caused by its slanted sidewalls. Electromagnetic simulations, including 3D finite-difference time-domain (FDTD) simulations, show a waveguide (mesa) width of 2 μm to be ideal in terms of mode coupling efficiency and overall absorption, as well as tolerance for transfer-printing alignment. Introducing a taper in the non-absorbing InP sub-contact layer might reduce the reflections at the high index contact transition. However, eigenmode expansion (EME) propagation simulations showed little to no improvement in the amount of light reflected if a taper were introduced. The simulations showed that by intelligently choosing the waveguide width and coupon separation, reflections are very low. The simulations of the optimized design show reflections below 1%. Photodiodes with three different waveguide lengths (12, 16, and 20 μm) were designed as a trade-off between the absorption length and increased junction capacitance. From simulations, it is expected that already more than 80% of the optical power is absorbed after 12 μm, and over 90% at 16 μm.
The UTC PD is made entirely on a III–V source wafer [ Fig. 2(a) ]. This process includes two metal depositions for anode and cathode contacts. The metal stack consists of 10 nm Ti, promoting the adhesion to the InGaAs layers, as well as 40 nm Pt, to prevent diffusion of the top Au layer. A self-aligned 2 μm-wide waveguide mesa is etched down to the cathode contact layer, followed by a device etch down to the release layer using a SiN hard mask [ Fig. 2(b) ].
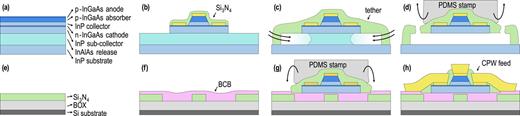
Fabrication flow: (a) epitaxial growth of the source III–V wafer. (b) Creation of a diode by etching the mesa, deposition of anode and cathode contacts, and SiN passivation. (c) After tethering the diode to the substrate with a new of layer SiN, the release layer is etched away. (d) This suspended coupon is then picked up by a polydimethylsiloxane (PDMS) stamp of the micro-transfer-printer. (e) The silicon target wafer with 300 nm SiN on top of the BOX. (f) Waveguides are created, and a thin layer of benzocyclobutene (BCB) is spin-coated. (g) The coupon is then printed on top of the waveguide and (h) post-processed to create electrical contacts.
To make transfer-printing possible, these diodes need to be converted into diode coupons, i.e., pickable chiplets. To do so, the release layer is first etched anisotropically with a padding of a few micrometer around the diode. A SiN encapsulation layer is deposited, and tethers that support the diode are patterned and etched within. The thickness of this layer is 500 nm, which yields a layer strong enough to support the coupon but still weak enough to break during the pick-up step of transfer-printing. Finally, the remainder of the InAlAs release layer is underetched isotropically using a chilled FeCl 3 solution, resulting in a suspended coupon [ Fig. 2(c) ].
Next, a photonic integrated circuit (PIC) was designed and fabricated on a SiN platform using in-house e-beam lithography. The material stack of the silicon target wafer consists of 3.3 μm-thick buried oxide (BOX) and 300 nm-thick SiN [ Fig. 2(e) ]. The design features an array of simple printing sites. For easy coupling, each photodiode is fed by a 0.5 mm long waveguide connected to a focused grating coupler. A thin layer of benzocyclobutene (BCB) is spin-coated on the sample, which makes the adhesion better during transfer-printing and fixates the coupon after curing [ Fig. 2(f) ].
The coupon is then transfer-printed by picking it up from the source wafer [ Fig. 2(d) ] and printing it on the target wafer [ Fig. 2(g) ] using a dedicated flexible stamp, made out of polydimethylsiloxane (PDMS). As a final step after transfer-printing, vias are etched in the SiN-layer and a coplanar waveguide (CPW) of 100 μm is deposited. This allows us to probe the photodiode electrically.
1. Passives
The SiN waveguides were verified to have losses below 1 dB/cm. Although this is still very low, waveguide etching in an industrial cleanroom (as compared to our research-grade tools) and only applying the BCB adhesion layer on a limited section of the waveguides (by locally opening up the top cladding layer) would further improve this. The grating couplers show an insertion loss of 9 dB at 1550 nm. These high losses can be attributed to the design of this coupler, as well as the BCB layer, which shifts the coupler’s central wavelength away from 1550 nm. As the grating coupler is not an intrinsic part of the photodetector and coupling losses could easily be lowered using an edge coupler, we will, from now on, consider the on-chip power.
2. Responsivity
The responsivity of the photodiodes at a wavelength of 1550 nm is measured to be 0.3 A/W. This responsivity was verified to be constant for bias voltages ranging between −2.5 and 0 V, as well at low and high power levels, up to multiple milliwatts on-chip power. This results in a (waveguide-referenced) external quantum efficiency (EQE) of 23%.
Although photodiodes with different waveguide lengths—12, 16, and 20 μm—were made, no significant correlation with an increased responsivity is found. It is believed that the excess light is absorbed in areas not contributing to the photocurrent, such as the metal contacts. Hence, increasing the length of the waveguide-coupled UTC PD is not beneficial.
3. Dark current
The dark current equals to 10 and 5 nA at −1.0 and −0.5 V biases, respectively. Two clear distinct regions in the dark current response can be observed in Fig. 3 : a relatively flat region down to −1 V bias, and a strong increase beyond −1 V bias. In the former region, the Shockley–Read–Hall (SRH) recombination, assisted by traps, is the main contribution to the dark current, whereas in the latter, band-to-band tunneling is dominating. 12 We believe the dark current density, which is in the order of magnitude of 1 mA/cm 2 , can be attributed to the surface leakage current caused by the mesa sidewall passivation.
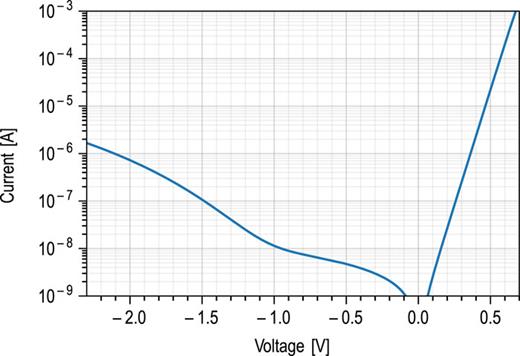
The dark current for a PD with a 2 × 16 μm 2 active area is below 10 nA for a bias voltage above −1 V.
4. Saturation
The 1 dB saturation point is reached at 4.6 mA photocurrent for −1 V bias with a RF power output of −4.1 dBm at 100 GHz as shown in Fig. 4 . This is also very close to the thermal breakdown current. At zero bias, the saturation is reached at 2.8 mA with an output power of −9.7 dBm at 100 GHz. The effective resistance r eff , given by P R F / I phot 2 , serves as a good measure for the photocurrent to RF conversion efficiency, independent of the optical power. 10 These diodes show a high efficiency with r eff going up to 20 Ω.
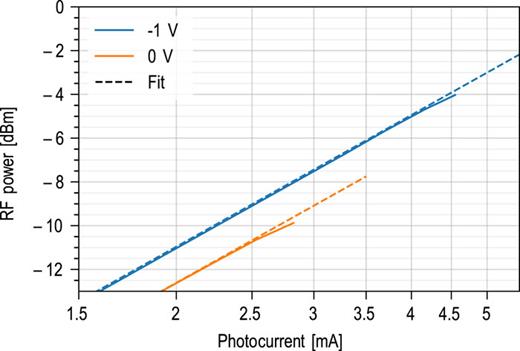
RF power at 100 GHz. The 1 dB saturation current for a PD with a 2 × 16 μm 2 active area is above 4.6 mA at −1 V bias and 2.8 mA at zero bias.
5. Impedance
The impedance of the photodiodes was measured with a vector network analyzer (VNA) up to 67 GHz. After de-embedding the results with a basic open–short calibration, a very good fit (R 2 > 0.99) with a series RC circuit could be made. It showed a junction capacitance of 18.5, 25, and 31 fF for PDs with a length of 12, 16, and 20 μm, respectively. This is a unit capacitance of 0.8 fF/μm 2 , slightly below the expected 1 fF/μm 2 for an InP collector of 100 nm. At zero-bias, the junction capacitance is about 10% higher. The series resistance is very low, varying between 4 and 10 Ω.
6. Frequency response
The frequency response of the photodiodes was measured using a set of power detectors. The lower frequency range (0–110 GHz) was measured using a coaxial probe, bias-tee, and thermal power meter (R&S NRP-Z58). The higher frequency ranges were measured with a calorimeter-style power meter (Erickson PM5) and two waveguide probes with an integrated bias-tee: a WR5-probe (140–220 GHz) and a WR3-probe (220–325 GHz). The results were corrected for probe, bias-tee, and THz-waveguide losses, as provided in the datasheets of the suppliers.
A photodiode with an active area of 2 × 12 μm 2 showed a 3-dB bandwidth of 155 GHz at −1 V bias, which is faster than any state-of-the-art photodiode heterogeneously integrated on SiN. 7,10 At zero bias, a bandwidth of 135 GHz is obtained, which is even higher than the best silicon-on-insulator (SOI) counterparts. 4 It should be noted that the 135 GHz-bandwidth is based on the interpolated data, as can be seen in the normalized frequency response ( Fig. 5 ).
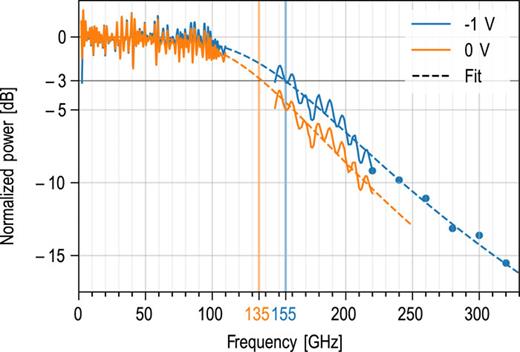
The 3-dB bandwidth for a PD with a 2 × 12 µ m 2 active area is 155 GHz at −1 V bias and 135 GHz at zero bias, both with a photocurrent of 1 mA.
Due to the thin layer thickness and high electron mobility in the absorption region, the bandwidth is mostly limited by the RC time constant (f RC ). 13 If we take into account the extracted junction capacitance C j of 18.5 fF, the series resistance R s of 4 Ω, and the 50 Ω impedance of the measurement equipment, the calculated f RC equals 159 GHz. The transit-time cut-off frequency (f TT ) is calculated to be 424 GHz for an estimated electron drift velocity of 3.5 × 10 7 cm/s in the 150-nm thick absorber. This results in an overall 3-dB bandwidth of 146 GHz, which is very close to the measured result. This small difference is attributed to the uncertainty on the effective electron velocity in the absorber and the possible inductive effects of the CPW contacts. At zero bias, the calculated RC-limit is 145 GHz, implying a small additional decrease in the transit-time bandwidth as well.
To demonstrate the capabilities of this photodiode at terahertz frequencies, a data experiment with a carrier frequency of around 300 GHz is set up ( Fig. 6 ). Two C-band laser lines, of which one is modulated by a Mach–Zehnder modulator (MZM), are combined and fed in the photonic integrated circuit (PIC). The mixed terahertz signal is then coupled out of the PIC by a ground–signal–ground (GSG) probe. Here, no electrical mixers at the transmit side are used and photomixing directly happens in the photodiode. This signal is then transmitted back-to-back (B2B), i.e., over a short WR-3 waveguide channel without additional attenuation. At the receiver side, the terahertz signal is down-converted using a Schottky-based diode mixer, post-amplified by 29 dB, and sampled by a real-time oscilloscope. A linear equalizer compensates for the dispersion introduced by the Schottky-based mixer: both its deterministic RF response and IF frequency dependence are compensated for. However, no pre-compensation is applied at the transmit side. Hence, the remaining distortion is due to the uncompensated roll-off behavior of the UTC-PD at around 280 GHz.
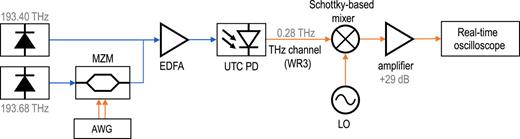
In this experimental setup, two separate lasers are used for photomixing. One line is modulated using a MZM, before being combined and amplified by an EDFA. The light is coupled into the PIC using a vertical grating coupler, and the mixed terahertz signal is then transferred over a WR3 waveguide channel before down-mixing, post-amplification, and sampling.
The performance of this THz-link was verified, and data rates beyond 100 Gbit/s were achieved with low error vector magnitudes (EVMs). All further values for the EVM are referenced to the peak constellation power. These high data rates were achieved using multiple combinations of constellation formats, e.g., 16-point quadrature amplitude modulation (16-QAM) at 35 GBd (140 Gbit/s) and 32-QAM at 25 GBd (125 Gbit/s). An optical input of 11 dBm was used, resulting in a photocurrent of 2.80 mA and RF-power of about −20 dBm (10 μW) at −1 V bias. At zero bias, the photocurrent is 2.65 mA, which is slightly lower due to the reduced bandwidth. As shown in Fig. 7 , a low EVM was achieved with a very small penalty for the absence of a bias voltage (zero bias). When using 16-QAM at 35 GBd (−1 V), the EVM rises by 0.7%, from 11.2% to 11.9%. Meanwhile, when using 32-QAM at 25 GBd (−1 V), the EVM only increased by 0.5%, from 7.0% to 7.5%.
![uni travelling carrier photodiode FIG. 7. A clear distinction between constellation points is visible at high data rates of 140 Gbit/s [(a) and (b)] and 125 Gbit/s [(c) and (d)], with a very small difference between −1 and 0 V biases. (a) 16-QAM at 35 GBd (−1 V) with an EVM of 11.2%. (b) 16-QAM at 35 GBd (0 V) with an EVM of 11.9%. (c) 32-QAM at 25 GBd (−1 V) with an EVM of 7.0%. (d) 32-QAM at 25 GBd (0 V) with an EVM of 7.5%.](https://aipp.silverchair-cdn.com/aipp/content_public/journal/app/8/1/10.1063_5.0119244/1/m_016104_1_f7.jpeg?Expires=1714741960&Signature=3Tjp5OQHX7~lR~HS-RqntKJjXKX7eDfCh5qWHrro7Q9LL0BZQeEJfu5a9slmLS5ZfVwUo9EoJST0rEM9NJfbdLlGuDqAw7h8nhVsFpzBvxeqs5ZrqDRfHqHfghNAupQeJexODOu7xwiWecwFlBCqjNKxCYvqK2sACiN4ssJLa6wClkM7jC~R7PihoeqdfvV33VuWRZmDM8d3G3c~43w7u3gQchZkFuosOW9eQmDD4a7QbYIsYkLInpdkLJ9BXeKG4SiXKfaEnLDMw4lfM2PUw980-v~Q1TNBuch-1dBrrqrizbwepBy55qvCRMCprj6hZL~TUm5NXi9sElOFei0~KQ__&Key-Pair-Id=APKAIE5G5CRDK6RD3PGA)
A clear distinction between constellation points is visible at high data rates of 140 Gbit/s [(a) and (b)] and 125 Gbit/s [(c) and (d)], with a very small difference between −1 and 0 V biases. (a) 16-QAM at 35 GBd (−1 V) with an EVM of 11.2%. (b) 16-QAM at 35 GBd (0 V) with an EVM of 11.9%. (c) 32-QAM at 25 GBd (−1 V) with an EVM of 7.0%. (d) 32-QAM at 25 GBd (0 V) with an EVM of 7.5%.
A second series of experiments was performed to asses the influence of the photocurrent level on the EVM. For a consistent result, this was done at a lower symbol rate of 4 GBd to avoid any influence of other bandwidth limiting devices in the setup. Figure 8 shows the EVM for a 4 GBd transmission. The photocurrent is limited to 1 mA as this was the maximal photocurrent achievable with the Thorlabs EDFA100P erbium-doped fiber amplifier (EDFA), which showed lower noise levels than the higher-powered Manlight EDFA. For an increased power, the EVM decreases according to an inversely-quadratic fit. This demonstrates that the EVM is noise limited and no linearity effects occur at 1 mA.
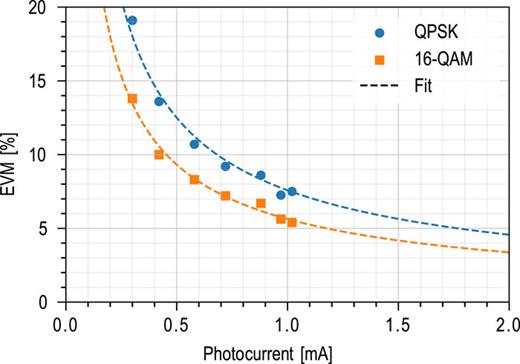
The EVM for a 4 GBd transmission shows a clear inversely-quadratic fit with no deterioration for increased photocurrents.
Waveguide-coupled UTC photodiodes can be integrated on a SiN-platform by means of micro-transfer-printing. This approach allows for the separate fabrication of the III–V chiplet and SiN PIC, ensures an efficient material usage, and delivers high-performance waveguide-coupled photodetectors. The demonstrated UTC PDs show a responsivity of 0.3 A/W, a low dark current below 10 nA (> −1 V bias), high bandwidths of 155 GHz at −1 V bias and 135 GHz at zero bias, and a saturation current of above 4.5 mA. We have showcased that this device can be used at terahertz frequencies (300 GHz) and supports data rates beyond 100 Gbit/s with a low EVM. To the best of our knowledge, this is the fastest photodiode on SiN and the fastest zero-bias photodiode cross-platform.
The authors have no conflicts to disclose.
Dennis Maes : Conceptualization (lead); Data curation (lead); Formal analysis (lead); Investigation (lead); Methodology (lead); Software (lead); Validation (lead); Visualization (lead); Writing – original draft (lead); Writing – review & editing (lead). Sam Lemey : Funding acquisition (equal); Supervision (equal); Writing – review & editing (equal). Gunther Roelkens : Supervision (supporting); Writing – review & editing (equal). Mohammed Zaknoune : Methodology (supporting); Writing – review & editing (supporting). Vanessa Avramovic : Methodology (supporting). Etienne Okada : Methodology (supporting). Pascal Szriftgiser : Methodology (supporting). Emilien Peytavit : Investigation (equal); Methodology (equal); Writing – review & editing (supporting). Guillaume Ducournau : Investigation (equal); Methodology (equal); Writing – review & editing (supporting). Bart Kuyken : Funding acquisition (equal); Supervision (equal); Writing – review & editing (equal).
The data that support the findings of this study are available from the corresponding author upon reasonable request.
Citing articles via
Submit your article.
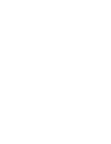
Sign up for alerts
- Online ISSN 2378-0967
- For Researchers
- For Librarians
- For Advertisers
- Our Publishing Partners
- Physics Today
- Conference Proceedings
- Special Topics
pubs.aip.org
- Privacy Policy
- Terms of Use
Connect with AIP Publishing
This feature is available to subscribers only.
Sign In or Create an Account
Thank you for visiting nature.com. You are using a browser version with limited support for CSS. To obtain the best experience, we recommend you use a more up to date browser (or turn off compatibility mode in Internet Explorer). In the meantime, to ensure continued support, we are displaying the site without styles and JavaScript.
- View all journals
- My Account Login
- Explore content
- About the journal
- Publish with us
- Sign up for alerts
- Open access
- Published: 09 June 2016
High-responsivity vertical-illumination Si/Ge uni-traveling-carrier photodiodes based on silicon-on-insulator substrate
- Chong Li 1 ,
- ChunLai Xue 2 ,
- Zhi Liu 2 ,
- Hui Cong 2 ,
- Buwen Cheng 2 ,
- Zonghai Hu 3 ,
- Xia Guo 1 , 3 &
- Wuming Liu 4
Scientific Reports volume 6 , Article number: 27743 ( 2016 ) Cite this article
3883 Accesses
27 Citations
2 Altmetric
Metrics details
- Electronics, photonics and device physics
- Silicon photonics
Si/Ge uni-traveling carrier photodiodes exhibit higher output current when space-charge effect is overcome and the thermal effects is suppressed. High current is beneficial for increasing the dynamic range of various microwave photonic systems and simplifying high-bit-rate digital receivers in many applications. From the point of view of packaging, detectors with vertical-illumination configuration can be easily handled by pick-and-place tools and are a popular choice for making photo-receiver modules. However, vertical-illumination Si/Ge uni-traveling carrier (UTC) devices suffer from inter-constraint between high speed and high responsivity. Here, we report a high responsivity vertical-illumination Si/Ge UTC photodiode based on a silicon-on-insulator substrate. When the transmission of the monolayer anti-reflection coating was maximum, the maximum absorption efficiency of the devices was 1.45 times greater than the silicon substrate owing to constructive interference. The Si/Ge UTC photodiode had a dominant responsivity at 1550 nm of 0.18 A/W, a 50% improvement even with a 25% thinner Ge absorption layer.
Similar content being viewed by others
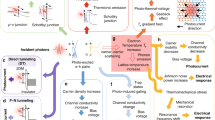
Silicon/2D-material photodetectors: from near-infrared to mid-infrared
Chaoyue Liu, Jingshu Guo, … Daoxin Dai
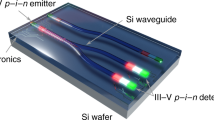
High-speed III-V nanowire photodetector monolithically integrated on Si
Svenja Mauthe, Yannick Baumgartner, … Kirsten E. Moselund
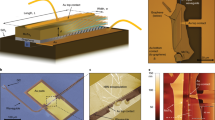
Waveguide-integrated van der Waals heterostructure photodetector at telecom wavelengths with high speed and high responsivity
Nikolaus Flöry, Ping Ma, … Lukas Novotny
Introduction
High-current photodiodes, which receive communication signals in the near-infrared range, are highly beneficial for increasing the dynamic range of various photonic systems 1 , 2 and simplifying high-bit-rate digital receivers 3 . The output radio-frequency signal level from these photodiodes can be increased with the response photocurrent. High-current photodiodes are particularly important components in optically-steered phased array antenna systems, because they can help the antenna to reduce its phase- and amplitude-matched electronic gain 4 , 5 , 6 . However, the conventional pin structure has a limitation in current density during high frequency operation, owing to the space-charge effect 7 , 8 .
The uni-traveling carrier (UTC) structure was designed to overcome the space-charge effect using a p-type doped absorption layer instead of a conventional intrinsic layer 9 , 10 , 11 , 12 , 13 , 14 . However, the photo-generated electrons diffuse from the p-type layer to the collection layer, resulting in a greatly reduce transit frequency. The reported transit frequency of typical UTC devices (with a 40 μm-diameter mesa and a 0.6 μm carrier diffusion length) approaches the RC frequency 9 , 15 . There are two general methods to increase the bandwidth. One is reducin the size of the device 16 , 17 , 18 , 19 . Unfortunately, smaller area of devices induces high thermal heating inside, which generates a negative feedback of the output power of these devices 10 , 20 . Monoatomic crystals such as Ge and Si have higher thermal conductivity than InGaAs and InP alloy materials 21 . Additionally, Si/Ge devices have great advantages in their compatibility with complementary metal-oxide-semiconductor (CMOS) technology and large-scale monolithic integration circuits, low cost and low power consumption 22 , 23 , 24 , 25 , 26 . Therefore, Si/Ge uni-traveling carrier photodiodes have dramatic practical potential for high-current output applications 19 , 27 . The other method is shortening the diffusion length 28 . However, the absorption efficiency decreases with the diffusion length, for the devices with the most commonly-used vertical-illumination configuration. Vertical-illumination configuration has great advantage on easily handling by pick-and-place tools and is a most popular choice for making photo-receiver modules 29 , 30 , 31 . To our knowledge, the first vertical-illumination Si/Ge UTC device was reported by M. Piels, who demonstrated a low thermal impedance of 520 K/W and a 1 dB saturation photocurrent of 20 mA. The responsivity of their device at 1550 nm was 0.12 A/W with a 0.8-μm-thick Ge absorption layer on silicon substrate 27 . Our group reported a 40 μm-diameter vertical-illumination Si/Ge UTC device with a high responsivity of 0.18 A/W but low bandwidth of 2.7 GHz 32 . Increasing the thickness of the Ge absorption layer could improve the responsivity 31 , but decrease the electron transit frequency and the response speed 34 . Therefore, it is a challenge to obtain both high responsivity and high speed at the same time in vertical-illumination Si/Ge UTC detectors.
Silicon-on-insulator (SOI) substrates have great advantages on the responsivity and bandwidth performance of Si/Ge photodiodes. First, the large difference in refractive index between the buried oxide layer (BOX) and the Si is beneficial in recycling transmission light back to the absorption layer, which is equivalent to extending the absorption length. This allows the absorption efficiency of the photodiodes to be increased without sacrificing the response speed. Second, high quality Ge film with low threading dislocation density (TDD) can be obtained on the SOI substrate by elastic deformation of the top silicon membrane and adapt to the lattice of the Ge hetero-epitaxial file, which could increase the carrier lifetime and decrease the non-radiative recombination rate, increasing the collection efficiency 33 , 34 , 35 , 36 . Therefore, the carrier collection efficiency of the device may be enhanced using a SOI substrate. Third, the use of a SOI substrate could reduce the parasitic capacitance of the device, which would result in a better frequency response performance 37 , 38 and a decrease in power loss 39 .
Here, we report a high-speed, high responsivity vertical-illumination Si/Ge UTC-PD based on a silicon-on-insulator (SOI) substrate. The silicon-on-insulator substrate was used to reflect transmission light for high absorption efficiency and to improve the lattice quality of the Ge epitaxial layer to increase the efficiency of photon-generated carrier collection. The absorption efficiency of the Ge-on-SOI UTC photodiode was found to vary periodically with the thicknesses of both the BOX and Si layers, owing to the interference between the incident light and the light reflected by the BOX layer of the SOI. Moreover, the maximum absorption efficiency of the devices on SOI was found to be 1.45 times greater than that of the silicon substrate and 2.3 times greater than the minimum absorption efficiency, with the maximum light transmission of the monolayer anti-reflection coating. We achieved a 3-dB bandwidth much larger than that in our previous work 33 by using a smaller device size of 15-μm-diameter. Meamwhile the thermal effect usually more serious in smaller devices was suppresed by using a large second meas electrde and the 1-dB compression current was 16.2 mA at 3 GH, which is similar to the 40-μm-diameter device at 1 GH 33 .
Structure and electric field
Expermentally, the open circle shaped top mesa electrode was desighed to improve the success rate of lift-off. And the large second mesa electrode with a 10-μm-width as shown in the inset of Fig. 1(a) was used to supress the thermal effect to ensure a high compress current. Figure 1(a) shows a cross-sectional schematic view of a Si/Ge UTC photodiode based on a commercially available SOI substrate with 1.0-μm-thick n-doped Si and 2-μm-thick BOX layers. A step gradient doped profile was employed, which enabled the generation of several regions with high local electric field to further decrease the transit time of the photo-generated electrons, as the red curve shown in Fig. 1(b) . A simulated band-gap diagram and electric field distribution at 0 V of such a device are illustrated in Fig. 1(b) by the blue and red curves, respectively, calculated after modifying the doped parameters according to the results of secondary ion mass spectrometry (SIMS) measurements. The built-in electric field was generated from the differences in the doped concentration. Each abrupt change in doped concentration corresponded to an electric field peak. The photon-generated electrons were accelerated in the Ge absorption layer and gained kinetic energy to pass through the Si/Ge heterojunction barrier under the action of the built-in electric field. A larger electric field and thus lower transit time can be obtained as compared to conventional linear-gradient-doped absorption layer 11 , 40 , 41 .
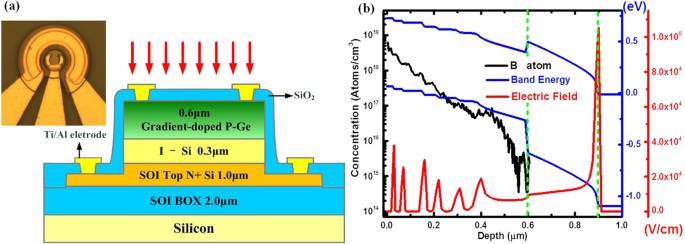
( a ) Cross-sectional schematic view of the reported Ge-on-SOI UTC photodetector and top view of a double-mesa structure of the Ge-on-SOI UTC photodetector. The substrate was SOI with a 1.0-μm-thick n-doped top Si film and a 2-μm-thick BOX layer, the collect layer was a 0.3-μm-thick intrinsic epitaxial silicon layer and the absorption layer was a 0.6-μm-thick epitaxial germanium layer with step gradient doping of B atoms. ( b ) The left black coordinate and curve show the doping concentration of B atoms in the Ge absorption layer step, as determined by SIMS. The etch step was nearly 0.05 μm wide, resulting in six high local electric fields to accelerate the photon-generated electrons and shorten the transmit time. In the simulation, the doping concentration in absorption layer decreased form 5 × 10 19 /cm 3 to 2 × 10 17 /cm 3 , which was modified according to the doping parameters of SIMS measurements. The right red and blue coordinates show the electric field and band energy of our devices without bias, respectively. The peak value and width of the six local electric fields were determined by the doping concentration around the step interfaces. According to our simulation, six step gradients in the absorption layer enabled a maximum potential difference across the layer.
Responsivity characterization
The responsivity of a vertical-illumination photodiode is limited mainly by a combination of three factors: (1) the coupling efficiency determined by the top anti-reflection coating, (2) the collection efficiency of the photon-generated carriers 42 , (3) the absorption efficiency of the Ge layer. Generally, only the light coupled into the absorber ( P 0 ) can be converted into electron-hole pairs. To maximize the coupled light and minimize the antireflection loss, the thickness of the top anti-reflection coating should be N·(λ/4n), due to destructive coherence inside the coating. In our device, N = 3, n is the refractive index of the coating (SiO 2 : ~1.47) 43 and the transmission is about 0.94. The carrier collection efficiency of Si/Ge UTC photodiodes is mainly determined by the design of the electric field inside the devices and by the recombination caused by defects inside the Ge layer and at the hetero-interface 44 , 45 . Absorption efficiency is generally dependent on the absorption coefficient and absorption length. The absorption coefficient of Ge at 1550 nm could be significantly affected by the tensile-strain and generally changed from 840 /cm (0% tensile-strain) to 4570 /cm (0.25% tensile-strain) 46 .
Assuming a 100% carrier collection efficiency, the absorption length is generally equal to the thickness of the absorber. Therefore, the power inside the absorption layer P ab can be expressed by P ab = P 0 ( 1 − e − aD ), where α is the absorption coefficient of the absorber and D is thickness of the absorber. However, the introduction of the SOI substrate was expected to cause recycling of the transmission light and improve the light absorption length and efficiency by the reflection of the substrate transmission light. The new absorption power is:
where R ref is the reflection coefficient of the SOI substrate and R ref is the reflection coefficient of the top coating film and Ge film.
A commercial finite-difference-time-domain (FDTD) simulation package was used to compare the detailed optical power distributions in the Si/Ge UTC photodiodes on the Si and SOI substrates with the same incident light, top anti-reflection coating and Ge layer, as shown in Fig. 2(a,b) . The scale bar is for the optical power. The optical power inside the Si bottom layer of the SOI substrate is obviously much lower than that in the silicon substrate. Therefore, the SOI substrate is more beneficial for higher light absorption by the Ge layer than the Si substrate.
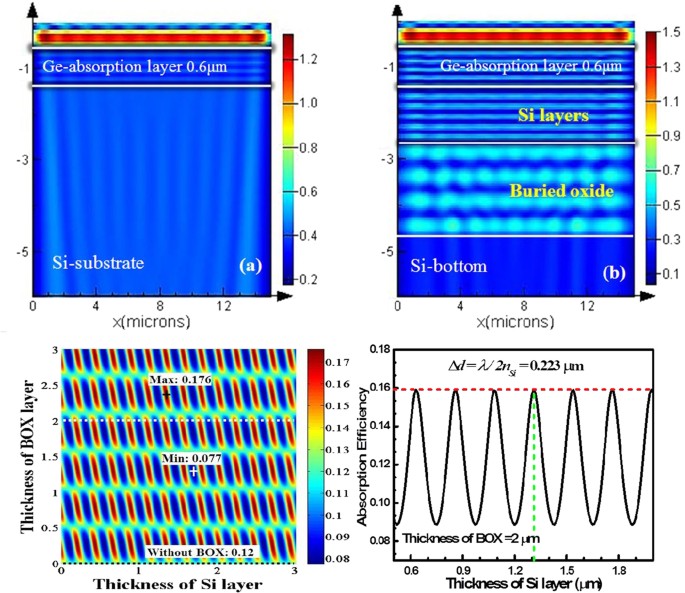
The wavelength of the incident light was 1550 nm and the absorption efficiency of the Ge material was 1000/cm.
Optical power distribution inside Si/Ge UTC devices grown on ( a ) Si substrate and ( b ) SOI substrate with the same imput light, same top anti-reflection coating and same Ge layer. The only difference between the two devices was the inserted 2-μm-thick BOX layer and the thickness of the silicon collection and contact layers was 1.3 μm. The scale bar illustrates the optical power. Because of the coherence effect between the incident light and reflected light of the BOX layer, the optical power inside the Ge, Si and BOX exhibited a periodic enhancement distribution. The period was determined by the refractive index and the wavelength of the incident light. The light inside the Si bottom layer of the SOI substrate and inside silicon substrate was the unemployed light of the devices. Obviously, that in the SOI was much lower than that in the silicon substrate. Thus, the SOI substrate was more beneficial for higher light absorption by Ge, compared with the Si substrate. When the transmission of the anti-reflection coating was maximum, ( c ) Relationship between the absorption efficiency of Ge-on-SOI UTC photodiode and the thicknesses of the BOX and silicon layers. Using these thicknesses of the BOX and silicon as ordinate and abscissa, respectively, the absorption efficiency is mapped in colored points in a two-dimensional coordinate plane. The bottom black dashed line represents the absorption efficiency of the devices without BOX layer, which was 0.12. The other two special points are the maximum of 0.176 with a 1.11-μm-thick Si layer and a 1.325-μm-thick BOX and the minimum of 0.077 with a 2.13-μm-thick Si layer and a 1.84-μm-thick BOX. ( d ) Relationship between the absorption efficiency and the thickness of the silicon layer, when the thickness of the BOX is the general commercial value of 2 μm. The absorption efficiency changes periodically with the thickness of Si layer because of constructive interference. The period is T = λ/2n, which is 0.223 μm for the silicon layer and the maximum efficiency is 0.159 when the silicon thickness is 1.31 μm, which is close to the structural parameter of our device.
The relationship between the absorption efficiency and the thickness of the BOX and silicon layer was analysed and calculated by the scattering matrix methods using Matrix Laboratory (MATLAB). When the transmission of the anti-reflection coating was maximum, the absorption efficiency varied periodically with the thickness of the substrates, as shown in Fig. 2 (c) . The SOI substrate is a compliant substrate, which could further release the built-in strain 33 , 52 , 47 . Therefore, it is supposed that the theoretical absorption coefficient at 1550 nm of our epitaxial Ge is 1000/cm with a lower than 0.13% tensile-strain, which is the tensile-strain of the Ge epitaxial layer on silicon substrate in our laboratory 48 , 49 , 50 . The absorption efficiency of the device was 0.121 with a 0.8-μm-thick coating film without BOX. The absorption efficiency increased to 0.176 with a 1.11-μm-thick Si layer and a 1.325-μm-thick BOX. However, the general thickness of the BOX layer in commercial SOI substrates is 2 μm and the absorption efficiency varied periodically with the thicknesses of Si shown in Fig. 2(d) . Because of the constructive interference between the reflected and input waves, the thickness period ( T ) is T = λ/2n, where λ is the input wavelength and n is the refractive index of the transmission media. In our device, the silicon thickness cycle was 0.223 μm with a Si thickness of 1.3 μm. Thus, the theoretical maximal absorption efficiency is about 0.157 and the ideal responsivity is 0.196 A/W with 100% photon-generated carrier collection efficiency and a 1000 /cm absorption coefficient at 1550 nm.
The experimentally determined device current for the 15-μm-diameter photodiodes, without illumination and with the normally incident light on the top surface, are shown in Fig. 3 . The dark current was 58 nA under a reverse bias of 1 V, which corresponds to a current density of 96.3 mA/cm 2 . The minimum dark current density was approximately 61.9 mA/cm 2 of the 40-μm-diameter device at −1 V. The dark current could be further reduced by appropriate thermal processing to decrease the threading dislocation density around the Si/Ge interface 51 , 52 ,passivation, or application of a guard-ring around the sidewall.
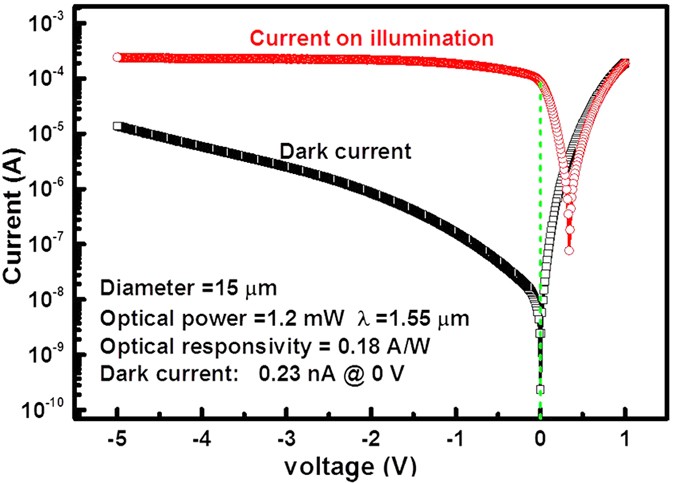
Current-voltage characteristics of the 15-μm-diameter device without illumination and under laser irradiation with an input optical power of 1.2 mW at 1550 nm.
The dark current without bias was 0.23 nA. When the reverse bias was increased to 1 V, the dark current rose to 58 nA, corresponding with a current density of 96.3 mA/cm. The optical responsivity was 0.18 A/W at 1550 nm. The dashed line indicates that there was a saturation of the optical responsivity values at 0 V bias, which indicates that this photodetector configuration allowed nearly complete photo-generated carrier collection without bias.
At a reverse bias of 1 V, the optical responsivity was 0.18 A/W at 1550 nm with a 0.6-μm-thick Ge layer, 50% higher than in the previously reported Si/Ge UTC devices ( R = 0.12 A/W with a 0.8-μm-thick Ge layer) 27 . In Fig. 4 , The photocurrent is flat over a wide range of reverse bias voltage and the optical currents around zero bias voltage show that a built-in electric field is already established within the p-type Ge layer and Si layer without applying a bia 53 . Because of the carrier recombination, this measured responsivity is little lower than the theoretical results.
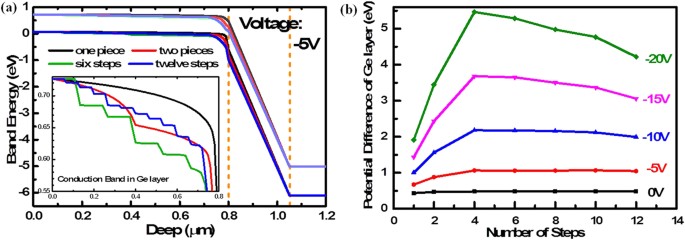
( a ) The band energy of the Si/Ge UTC photodiodes with a linear-gradient-doped (one piece), linear-gradient-doped with a step (two pieces), 6-step-and 12-step- gradient-doped Ge absorption layer at -5 V bias. the inset figure show the conduction band in Ge layer. The potential difference of the linear gradient doped Ge layer is lower than the step-gradient-doped structure. ( b ) The potential difference of Ge layer with one step, 2-step-, 4-step-, 6-step-, 8-step-, 10-step- and 12-step-gradient-doped at different biases. The peak potential difference is 0.48 eV at 0 V with 4-step-gradient-doped, approximate 8% of the total potential difference of the devices. However, when the bias voltage increased to −20 V, the potential difference of Ge layer is 5.46 eV, almost 21% of the device potential difference. Actually, limited by the technology of the in-situ doped epitaxial, the Ge layer of our device is six steps gradient doped.
3-dB bandwidth characterization
The bandwidth of common photodiode is limited mainly by the resistor–capacitor (RC) bandwidth ( f RC ) and the carrier transit-time-limited bandwidth ( f t ) in the active region 54 . Particularly, for UTC devices, the transfer time of electrons in the p-type absorption layer is more important than the RC time constant, owing to their low diffusion velocity 16 . Based on the principle of conservation of energy, the electrical potential difference across the absorption layer determines the change in kinetic energy of the carriers. A high kinetic energy in the absorber could shorten the transfer time of the electrons. Based on the direct relationship between the doping difference across the doped junction and the electrical potential difference, a step gradient-doped region was introduced in the absorption layer to increase the potential difference across the Ge layer. Figure 4(a) shows the band energy of the devices with a linear-gradient-doped, linear-gradient-doped with 1-step, 6-step-and 12-step- gradient-doped absorption layer. The doping profile is uniform within each step region. The electrical potential difference in the step-gradient doped layer is higher than that in the conventional linear gradient doped layer. We also calculated the potential differences of Ge layer with one step, 2-step-, 4-step-, 6-step-, 8-step-, 10-step- and 12-step- gradient-doped at different bias, shown in Fig. 4(b) . The maximal value of the potential difference value can be achieved with a 4-step-gradiant-doped Ge layer. However, limited by the technology of the in-situ doped epitaxy, the Ge layer of our device is six steps gradient doped. Therefore, we can assume that the photo-generated electrons drifted with saturation velocity ( v s ) in the p-type absorption layer of our devices owing to the high potential drop across the absorber and then cross the collection layer with thermionic emission velocity ( v th ), because of the effect of the velocity overshoot 9 . Then, the carrier transit frequency can be approximated by the following equation:
where v th is the thermionic emission velocity and v s is the saturation velocity.
Moreover, there are no depletion or depletion-layer capacitance in absorption layer due to same material, same bandgap and same doping type around the step-gradient doped interfaces. The parasitic capacitance, resulting from leak current and threading dislocation in the Ge epitaxial layer, is small enough to be ignored compared with the junction capacitance. Therefore and f RC can be approximated using:
where W c is the collection layer thickness, W a is the absorption layer thickness, d is the thickness of depletion region, D is the mesa diameter, R L is the load resistance (50 Ω in this case), R S is the series resistance and ε and ε 0 are the relative and vacuum permittivity, respectively. Series resistance arises from the resistance of the contacts and the resistance of the un-depleted region, which could be inferred by the forward I-V characteristic curves. The series resistance is inversely proportional to the area of the device, approximately equal to the slope of the I-V curve at positive bias 55 . Here, the used series resistance of the device is about 100 Ω, which was the measured resistance of the 40 μm-diameter device 33 . The theoretical values of f 3 dB with various diameters are shown in Fig. 5(a) . The consistency between the experimental and theoretical results showed that the step gradient-doped design was able to make the carriers drift in the absorber with a saturation velocity and efficiently increase the transit frequency of the UTC photodiodes. Because the series resistance is inversely proportional to the area of the device, the actual bandwidth of the 15-μm-diameter device is little lower than the calculated value, as shown in Fig. 5(b) . The bandwidth of our photodiodes was mainly limited by the high contact resistance of n-type Si layer, which were measured to be .1.75 × 10 −3 Ω·cm 2 and 2~3 orders of magnitude more than the normal contact resistance 56 . Specific contact resistance is a function of the barrier height, doping concentration and temperature for a fixed semiconductor material. Therefore, some approaches, such as Al contact metal instead of Ti/Al, ions re-implantation in metal contact region and wet etching before metallizing to get rid of the etching residue or oxide film on the semiconductor, could significantly reduce the series resistance of our photodiode. Theoretically, the 3-dB bandwidth of our 15 μm-diameter could be as high as 51 GHz with a 1 Ω series resistance about 30 GHz higher bandwidth than previously reported bandwidth 33 . More work to improve this bandwidth is underway. Additional, the parasitic capacitance and series resistance of the SOI substrate is lower than silicon substrate. Therefore, the bandwidth of devices based on SOI should be higher than based on silicon substrate, theoretically.
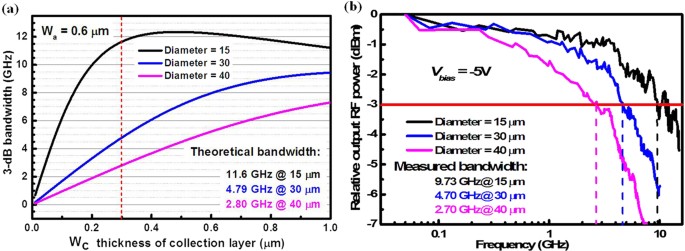
Frequency responses for UTC photodiodes with diameters of 15, 30 and 40 μm under 1550 nm incident light: (a ) theoretically predicted values for a series resistance of 100 Ω, which was measured using the slope of the I-V curve at the positive bias of 0.26 V and on the assumption that the photo-generated electrons traveled across the p-type absorption layer by drifting with saturation velocity ( v s ) and across the collect layer by drifting with thermionic emission velocity ( v th ). ( b ) The 3-dB bandwidth was measured with a vector network analyser with a bias of −5 V. The theoretical f 3 dB values are almost consistent with the experimental results except for the 15-μm-diameter device, whose difference may have resulted from the higher series resistance of smaller size devices caused by the complicated fabrication processes.
Saturation characterization
The device saturation current was obtained using large signal measurements, as shown in Fig. 6 . A 100% modulation depth tone was fixed at 3 GHz. The 15-μm-diameter device exbibited a 3 dB bandwidth of 9.7 GHz. The 1-dB compression currents was 16.2 mA at reverse bias voltages of -6 V. The fixed modulation frequency of the 40-μm-diameter device in ref. 33 was 1 GHz. The a 3 dB bandwidth was 2.7 GHz and the saturation current was also 16.2 mA under −7 V bias. Three main effects limit the photocurrent saturation of the device: voltage drop and swing, thermal effect and space-charge effect 15 . The high modulation frequency could increase the voltage drop and the small area of device could enhance space-charge effect, which both decrease the compression current of the photodiode. However, the large second mesa electrode of the 15-μm-diameter device had great benefits on the thermal dissipation and low series resistance. Therefore, our 15-μm-diameter device have a similar -1-dB compression current yet with a higher modulation frequency, higher bandwidth and a lower working voltage, compared with the 40-μm-diameter device in ref. 33 . Moreover, The saturation of the Ge-on-SOI UTC photodiodes could be further improved by suppressing the thermal effects 56 , 57 by substrate thinning 27 and decreasing the thickness of the BOX layer.
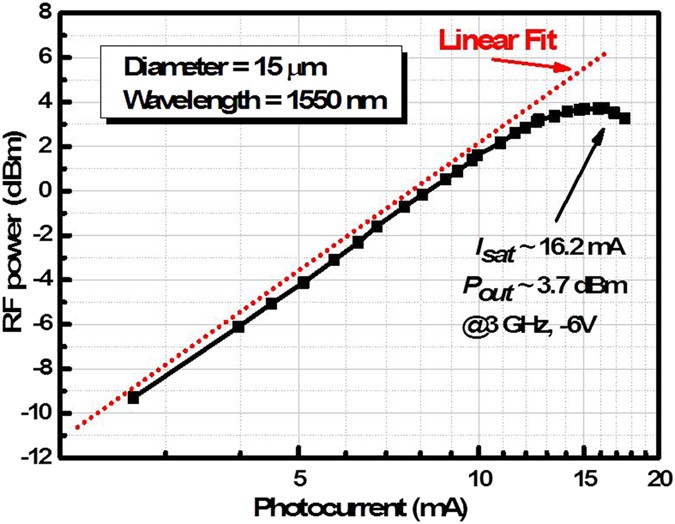
Results of large signal −1-dB compression photocurrent measurement for the 15-μm-diameter Si/Ge UTC photodiodes.
The incident light had a wavelength of 1550 nm, 100% modulation depth and modulation frequency fixed at 3 GHz. The saturation current was 16.2 mA at a reverse bias of 6 V and the output RF power was 3.7 dBmW. The saturation current and RF power were further increased with the reverse bias.
The demonstrated on-chip performance of the present high-responsivity vertical-illumination Si/Ge uni-traveling-carrier photodiodes paves the way for all kinds of vertical-illumination Si/Ge photodetectors with high responsivity and high-quality epitaxial germanium. It will also allow the realization of large-scale monolithic integrated microwave optoelectronic antenna systems with low cost and low power consumption. The use of silicon-on-insulator substrate for Si/Ge UTC photodiodes offers advantages in reflecting the light to increase the absorption efficiency of the input optical signal and improving the lattice quality of Ge epitaxial layer to increase the efficiency of photon generated carrier collection. Because of the constructive interference between the incident light and the light reflected by the buried oxide layer of the SOI, the maximum absorption efficiency of the devices on SOI substrate was 1.45 times greater than that obtained with silicon substrate and 2.3 times greater than the minimum absorption efficiency with a maximum transmission of the anti-reflection coating. The photodiodes showed a responsivity of 0.18 A/W at a wavelength of 1.55 μm, which is 50% higher than the previously reported Si/Ge UTC devices using Si substrates. Furthermore, use of a step gradient-doped absorber caused the carriers to drift with a saturation velocity in the absorber, which efficiently increased the transit frequency of the UTC photodiodes. As a result, the 3-dB bandwidth of the 15-μm-diameter device was improved to 9.72 GHz under a −5 V bias voltage. The 1-dB compression current of the device was 16.2 mA at 3 GHz. Hindered by the high series resistance and the area of the device, the performance of our device is not yet comparable to the more mature III/V devices. Nevertheless, this study introduces the great potential of Si/Ge UTC photodiodes to high-speed microwave photonic monolithic integrated applications.
Silicon and germanium film growth and characteristics
After the growth of the intrinsic Si layer at 750 °C by cold-wall ultra-high vacuum chemical vapour deposition on the SOI substrate using a source gas of pure Si 2 H 6 (UHV-CVD), a brief interruption was introduced to decrease the growth temperature to 290 °C for the growth of the 60-nm-thick p-doped Ge buffer layer. A 600-nm-thick boron-doped Ge layer was then grown on the top as the absorption layer at 600 °C using pure GeH 4 and diluted B 2 H 6 source gases. Six boron-doping concentration steps were made in the Ge absorption layer.
Fabrication and characterization of photodiodes
Circular Ge layer mesas for normal incidence Si/Ge UTC photodiodes with diameters ranging from 15 to 40 μm were defined by standard photolithography and inductively coupled plasma (ICP) etching. The second mesa was etched to the 2-μm-thick buried oxide layer. The double mesa layout significantly reduced the parasitic capacitance. Top and bottom contacts were lithographically defined on evaporated Ti/Al and a rapid-thermal-annealing (RTA) process was carried out for impurity activation. A passivation/antireflection coating was deposited by plasma enhanced chemical vapour deposition (PE-CVD). Windows for the metal contacts were opened by C 4 F 8 ICP etching. The metal pad was evaporated and lifted off. A micrograph of a photodiode with a 15-diameter top mesa is shown in Fig. 1(b) . The current-voltage characteristics of our device was measured using an Agilent B1500A semiconductor parameter analyser on a probe station at room temperature. The photocurrent-voltage characteristics were obtained under laser irradiation at a wavelength of 1550 nm with power of 1.2 mW.

Saturation measurements
The device saturation current was obtained using large signal measurements. A heterodyne technique using two free-running lasers at 1550 nm was used and a modulation index was ultimately obtained. A 100% modulation depth tone was fixed at −3 GHz for measurement of the 15-μm-diameter device.
Additional Information
How to cite this article : Li, C. et al . High-responsivity vertical-illumination Si/Ge uni-traveling-carrier photodiodes based on silicon-on-insulator substrate. Sci. Rep. 6 , 27743; doi: 10.1038/srep27743 (2016).
Cox, C., Ackerman, E., Helkey, R. & Betts, G. E. Techniques and performance of intensity-modulation direct-detection analog optical links. IEEE Trans. Microw. Theory Techn. 45, 1375–1383 (1997).
Article ADS Google Scholar
Nichols, L. T., Williams, K. J. & Esman, R. D. Optimizing the ultrawideband photonic link. IEEE Trans. Microw. Theory Techn . 45, 1384–1389 (1997).
Williams, K. J., Dennis, M. L., Duling III, I. N., Villarruel, C. & Esman, R. D. A simple high-speed high-output voltage digital receiver. IEEE Photon. Technol. Lett . 10, 588–590 (1998).
Ito, H., Nakajima, F., Furuta, T. & Ishibashi, T. Continuous THz-wave generation using antenna-integrated uni-travelling-carrier photodiodes. Semicond. Sci. Technol . 20, S191 (2005).
Article CAS ADS Google Scholar
Song, H. J. et al. Broadband-frequency-tunable sub-terahertz wave generation using an optical comb, AWGs, optical switches and a uni-traveling carrier photodiode for spectroscopic applications. IEEE/OSA J. Lightw. Technol . 26, 2521–2530 (2008).
Hisatake, S., Kim, J. Y., Ajito, K. & Nagatsuma, T. Self-heterodyne spectrometer using uni-traveling-carrier photodiodes for terahertz-wave generators and optoelectronic mixers. IEEE/OSA J. Lightw. Technol . 32, 3683–3689 (2014).
Williams, K. J., Esman, R. D. & Dagenais, M. Nonlinearities in pin microwave photodetectors. IEEE/OSA J. Lightw. Technol . 14, 84–96 (1996).
Hu, Y., Marks, B. S., Menyuk, C. R., Urick, V. J. & Williams, K. J. Modeling sources of nonlinearity in a simple pin photodetector. IEEE/OSA J. Lightw. Technol . 32, 3710–3720 (2014).
Ishibashi, T., Kodama, S., Shimizu, N. & Furuta, T. High-speed response of uni-traveling-carrier photodiodes. Jpn. J. Appl. Phys . 36, 6263 (1997).
Williams, K. J. & Esman, R. D. Design considerations for high-current photodetectors. IEEE/OSA J. Lightw. Technol ., 17, 1443 (1999).
Chtioui, M. et al. High-power high-linearity uni-traveling-carrier photodiodes for analog photonic links. IEEE Photon. Technol. Lett . 20, 202–204 (2008).
Shi, T., Xiong, B., Sun, C. & Luo, Y. Back-to-back UTC-PDs with high responsivity, high saturation current and wide bandwidth. IEEE Photon. Technol. Lett . 25, 136–139 (2013).
Li, N. et al. High-saturation-current charge-compensated InGaAs-InPuni-traveling-carrier photodiode. IEEE Photon. Technol. Lett . 16, 864–866 (2004).
Shi, J. W., Wu, Y. S., Wu, C. Y., Chiu, P. H. & Hong, C. C. High-speed, high-responsivity and high-power performance of near-ballistic uni-traveling-carrier photodiode at 1.55-μm wavelength. IEEE Photon. Technol. Lett . 17, 1929–1931 (2005).
Ishibashi, T., Furuta, T., Fushimi, H. & Ito, H. Photoresponse characteristics of uni-traveling-carrier photodiodes. Proc . SPIE 4283: Physics and Simulation of Optoelectronic Devices IX, 469, San Jose, CA, SPIE, doi: 10.1117/12.432597 (2001, July 9).
Zhou, Q. et al. High-power V-band InGaAs/InP photodiodes. IEEE Photon. Technol. Lett . 25, 907–909 (2013).
Shi, J. W., Kuo, F. M. & Bowers, J. E. Design and Analysis of Ultra-High-Speed Near-Ballistic Uni-Traveling-Carrier Photodiodes Under a 50-Load for High-Power Performance. IEEE Photon. Technol. Lett . 24, 533–535 (2012).
Rouvalis, E. et al. 170 GHz uni-traveling carrier photodiodes for InP-based photonic integrated circuits. Opt. Express 20, 20090–20095 (2012).
Article CAS ADS PubMed Google Scholar
Piels, M. & Bowers, J. E. 40 GHz Si/Ge uni-traveling carrier waveguide photodiode. IEEE/OSA J. Lightw. Technol . 32, 3502–3508 (2014).
Stievater, T. H. & Williams, K. J. Thermally induced nonlinearities in high-speed pin photodetectors. IEEE Photon. Technol. Lett . 16, 239–241 (2004).
Levinstein, M., Rumyantsev, S. & Shur, M. Handbook Series on Semiconductor Parameters , World Scientific 1, 2 (1999).
Google Scholar
Yang, Z. et al. Optical properties of Ge/Si quantum dot superlattices. Mater. Lett . 58, 3765–3768 (2004).
Article CAS Google Scholar
Wang, K. L. & Lynch, W. T. Scenarios of CMOS scaling. Proceedings of the IEEE International Conferenc on Solid-State and Integrated Circuit Technology (pp. 12–16): Beijing, IEEE, doi: 10.1109/ICSICT.1998.785772 (1998 October 21).
Han, G. et al. Silicon-based tunneling field-effect transistor with elevated germanium source formed on (110) silicon substrate. Appl. Phys. Lett . 98, 153502 (2011).
Article ADS CAS Google Scholar
Li, C., Chen, Y., Zhou, Z., Lai, H. & Chen, S. Enhanced photoluminescence of strained Ge with a δ-doping SiGe layer on silicon and silicon-on-insulator Appl. Phys. Lett . 95, 251102 (2009).
Kasper, E., Lyutovich, K., Bauer, M. & Oehme, M. New virtual substrate concept for vertical MOS transistors. Thin Solid Films 336, 319–322 (1998).
Piels, M. & Bowers, J. E. Si/Ge uni-traveling carrier photodetector. Opt. Express 20, 7488–7495 (2012).
Yu, H. Y. et al. High-efficiency pin photodetectors on selective-area-grown Ge for monolithic integration. IEEE Electron Device Letters 30, 1161–1163 (2009).
Wu, T. T., Chou, C. Y., Lee, M. C. M. & Na, N. A critically coupled Germanium photodetector under vertical illumination. Opt. Express 20, 29338–29346 (2012).
Oehme, M. et al. GeSn-on-Si normal incidence photodetectors with bandwidths more than 40 GHz. Opt. Express, 22, 839–846 (2014).
Article ADS CAS PubMed Google Scholar
Dehlinger, G. et al. High-speed germanium-on-SOI lateral PIN photodiodes. IEEE Photon. Technol. Lett . 16, 2547–2549 (2004).
Li, C. et al. High-responsivity and high-saturation-current Si/Ge uni-travelingcarrier photodetector . In SPIE Optical Engineering + Applications (pp. 960908–960908). International Society for Optics and Photonics, doi: 10.1117/12.2186742 (2015, August 28).
Yang, Z. et al. In situ relaxed Si1− xGex epitaxial layers with low threading dislocation densities grown on compliant Si-on-insulator substrates. J. Vac. Sci. Technol. B 16, 1489–1491 (1998).
Hobart, K. D. et al. Compliant substrates: a comparative study of the relaxation mechanisms of strained films bonded to high and low viscosity oxides. J. Electron. Mater . 29, 897–900 (2000).
Bandić, Z. Z., Bridger, P. M., Piquette, E. C. & McGill, T. C. Minority carrier diffusion length and lifetime in GaN. Appl. Phys. Lett . 72, 3166–3168 (1998).
Kurtz, A. D., Kulin, S. A. & Averbach, B. L. Effect of dislocations on the minority carrier lifetime in semiconductors. Phys. Rev . 101, 1285 (1956).
Su, L. T., Jacobs, J. B., Chung, J. & Antoniadis, D. A. Deep-submicrometer channel design in silicon-on-insulator (SOI) MOSFET’s. IEEE Electron Device Letters 15, 183–185 (1994).
Li, C. et al. High-Bandwidth and High-Responsivity Top-Illuminated Germanium Photodiodes for Optical Interconnection. IEEE Trans. Electron Devices 60, 1183–1187 (2013).
Green, W. M., Rooks, M. J., Sekaric, L. & Vlasov, Y. A. Ultra-compact, low RF power, 10 Gb/s silicon Mach-Zehnder modulator. Opt. Express 15, 17106–17113 (2007).
Article ADS PubMed Google Scholar
Pan, H., Li, Z., Beling, A. & Campbell, J. C. Measurement and modeling of high-linearity modified uni-traveling carrier photodiode with highly-doped absorber. Opt. Express 17, 20221–20226 (2009).
Jun, D. H., Jang, J. H., Adesida, I. & Song, J. I. Improved efficiency-bandwidth product of modified uni-traveling carrier photodiode structures using an undoped photo-absorption layer. Jpn. J. Appl. Phys . 45, 3475 (2006).
Fakharuddin, A., Ahmed, I., Khalidin, Z., Yusoff, M. M. & Jose, R. Channeling of electron transport to improve collection efficiency in mesoporous titanium dioxide dye sensitized solar cell stacks. Appl. Phys. Lett . 104, 053905 (2014).
Rosfjord, K. M. et al. Nanowire single-photon detector with an integrated optical cavity and anti-reflection coating. Opt. Express, 14, 527–534 (2006).
Kim, J. et al. Transparent conductor-embedding nanocones for selective emitters: optical and electrical improvements of Si solar cells. Scientific Reports 5, 9256 (2015).
Article CAS PubMed PubMed Central Google Scholar
Savuskan, V. et al. Single Photon Avalanche Diode Collection Efficiency Enhancement via Peripheral Well-Controlled Field. IEEE Trans. Electron Devices 62, 1939–1945 (2015).
Liu, J. et al. Tensile strained Ge pin photodetectors on Si platform for C and L band telecommunications. Appl. Phys. Lett . 87, 1110 (2005).
Jongthammanurak, S. et al. Large electro-optic effect in tensile strained Ge-on-Si films. Appl. Phys. Lett . 89, 161115 (2006).
Dash, W. C. & Newman, R. Intrinsic optical absorption in single-crystal germanium and silicon at 77 K and 300 K. Phys. Rev . 99, 1151 (1955).
Ishikawa, Y. et al. Strain-induced band gap shrinkage in Ge grown on Si substrate. Appl. Phys. Lett . 82, 2044–2046 (2003).
Zhi, L. et al. Effects of high temperature rapid thermal annealing on Ge films grown on Si (001) substrate. Chin. Phys. B 22, 116804 (2013).
Luan, H. C. et al. High-quality Ge epilayers on Si with low threading-dislocation densities. Appl. Phys. Lett . 75, 2909–2911 (1999).
Choi, D., Ge, Y., Harris, J. S., Cagnon, J. & Stemmer, S. Low surface roughness and threading dislocation density Ge growth on Si (001). J. Cryst. Growth 310, 4273–4279 (2008).
Liu, J. et al. High-performance, tensile-strained Ge pin photodetectors on a Si platform. Appl. Phys. Lett . 87, 103501 (2005).
Feng, D. et al. High-speed Ge photodetector monolithically integrated with large cross-section silicon-on-insulator waveguide. Appl. Phys. Lett . 95, 261105 (2009).
Sze, S. M. & Ng, K. K. Physics of semiconductor devices . John Wiley & Sons (2006).
Park, W., Hu, J., Jauregui, L. A., Ruan, X. & Chen, Y. P. Electrical and thermal conductivities of reduced graphene oxide/polystyrene composites. Appl. Phys. Lett . 104, 113101(2014).
Jauregui, L. A. et al. Thermal transport in graphene nanostructures: Experiments and simulations. Ecs Trans . 28, 73–83 (2010).
Download references
Acknowledgements
This work was supported in part by the National Natural Science Foundation of China under grant Nos 61505003, 61222501, 61335004, 11434015, 61378017and 61227902, by the National High-Technology Research and Development Program of China under grant No. 2012AA012202, by the Major State Basic Research Development Program of China under grant Nos 2013CB632103 and 2011CBA00608, by the Specialized Research Fund for the Doctoral Program of Higher Education of China under grant No. 20111103110019, NKBRSFC under grants Nos 2012CB821305, SKLQOQOD under grants No. KF201403 and SPRPCAS under grants No. XDB01020300.
Author information
Authors and affiliations.
Institute of Electronic Information and Control Engineering, Beijing University of Technology, 100124, Beijing, China
Chong Li & Xia Guo
State Key Laboratory on Integrated Optoelectronics, Institute of Semiconductors, Chinese Academy of Sciences, 100083, Beijing, China
ChunLai Xue, Zhi Liu, Hui Cong & Buwen Cheng
Minzu University of China, 100081, Beijing, China
Zonghai Hu & Xia Guo
Beijing National Laboratory for Condensed Matter Physics, Institute of Physics, Chinese Academy of Sciences, 100190, Beijing, China
You can also search for this author in PubMed Google Scholar
Contributions
C. Li, C. Xue and X. Guo conceived the study. Z. Liu conducted the sample-growth experiments. C. Li and H. Cong carried out the wafers growth experiments chips fabrication and characterization test. In the meanwhile, C. Li carried out the calculation and simulation. C. Xue, B. Cheng and X. Guo supervised the entire research project. C. Li, C. Xue, Z. Liu, B. Cheng, Z. Hu, X. Guo and W. Liu analysed the data. All authors discussed the results and wrote the manuscript.
Ethics declarations
Competing interests.
The authors declare no competing financial interests.
Rights and permissions
This work is licensed under a Creative Commons Attribution 4.0 International License. The images or other third party material in this article are included in the article’s Creative Commons license, unless indicated otherwise in the credit line; if the material is not included under the Creative Commons license, users will need to obtain permission from the license holder to reproduce the material. To view a copy of this license, visit http://creativecommons.org/licenses/by/4.0/
Reprints and permissions
About this article
Cite this article.
Li, C., Xue, C., Liu, Z. et al. High-responsivity vertical-illumination Si/Ge uni-traveling-carrier photodiodes based on silicon-on-insulator substrate. Sci Rep 6 , 27743 (2016). https://doi.org/10.1038/srep27743
Download citation
Received : 25 November 2015
Accepted : 24 May 2016
Published : 09 June 2016
DOI : https://doi.org/10.1038/srep27743
Share this article
Anyone you share the following link with will be able to read this content:
Sorry, a shareable link is not currently available for this article.
Provided by the Springer Nature SharedIt content-sharing initiative
This article is cited by
On the unusually high photosensitivity of two barrier structures.
- Ashok Vaseashta
- Surik Khudaverdyan
- Hayk Babajanyan
Applied Physics B (2023)
By submitting a comment you agree to abide by our Terms and Community Guidelines . If you find something abusive or that does not comply with our terms or guidelines please flag it as inappropriate.
Quick links
- Explore articles by subject
- Guide to authors
- Editorial policies
Sign up for the Nature Briefing newsletter — what matters in science, free to your inbox daily.


- Keep it simple - don't use too many different parameters.
- Example: (diode OR solid-state) AND laser [search contains "diode" or "solid-state" and laser]
- Example: (photons AND downconversion) - pump [search contains both "photons" and "downconversion" but not "pump"]
- Improve efficiency in your search by using wildcards.
- Asterisk ( * ) -- Example: "elect*" retrieves documents containing "electron," "electronic," and "electricity"
- Question mark (?) -- Example: "gr?y" retrieves documents containing "grey" or "gray"
- Use quotation marks " " around specific phrases where you want the entire phrase only.
- For best results, use the separate Authors field to search for author names.
- Use these formats for best results: Smith or J Smith
- Use a comma to separate multiple people: J Smith, RL Jones, Macarthur
- Note: Author names will be searched in the keywords field, also, but that may find papers where the person is mentioned, rather than papers they authored.
- pp. 884-889
- • https://doi.org/10.1364/OPTICA.6.000884
High-speed uni-traveling carrier photodiode for 2 μm wavelength application
Yaojiang Chen, Zhiyang Xie, Jian Huang, Zhuo Deng, and Baile Chen
Author Affiliations
Yaojiang Chen, 1, 2, 3 Zhiyang Xie, 1 Jian Huang, 1 Zhuo Deng, 1 and Baile Chen 1, *
1 School of Information Science and Technology, ShanghaiTech University, Shanghai 201210, China
2 Shanghai Institute of Microsystem and Information Technology, Chinese Academy of Sciences, Shanghai 200050, China
3 University of Chinese Academy of Sciences, Beijing 100049, China
* Corresponding author: [email protected]
- Share with Facebook
- Post on reddit
- Share with LinkedIn
- Add to Mendeley

- Share with WeChat
- Endnote (RIS)
- Citation alert
- Save article
- More Like This Dynamic model and bandwidth characterization of InGaAs/GaAsSb type-II quantum wells PIN photodiodes Yaojiang Chen, et al. Opt. Express 26 (26) 35034-35045 (2018) High-speed photo detection at two-micron-wavelength: technology enablement by GeSn/Ge... Shengqiang Xu, et al. Opt. Express 27 (4) 5798-5813 (2019) 0.5 Sb 0.5 /In 0.53 Ga 0.47 As type-II hybrid absorbers">Enhancement in speed and responsivity of uni-traveling carrier photodiodes with... Naseem, et al. Opt. Express 27 (11) 15495-15504 (2019)
- Optical components
- Optical systems
- Photodetectors
- Photonic bandgap fibers
- Short infrared
- Thulium-doped fiber amplifiers
- Original Manuscript: April 11, 2019
- Revised Manuscript: June 16, 2019
- Manuscript Accepted: June 17, 2019
- Published: July 12, 2019
- Back to Article
- INTRODUCTION
- DEVICE STRUCTURE AND FABRICATION
- MEASUREMENT RESULT
- References and links
- Figures ( 13 )
- Suppl. Mat. ( 1 )
- Tables ( 2 )
- Equations ( 2 )
- References ( 28 )
- Cited By ( 69 )
- Back to Top
Current optical communication systems operating at the 1.55 μm wavelength band may not be able to continually satisfy the growing demand on data capacity within the next few years. Opening a new spectral window around the 2 μm wavelength with recently developed hollow-core photonic bandgap fiber and a thulium-doped fiber amplifier is a promising solution to increase transmission capacity due to the low-loss and wide-bandwidth properties of these components at this wavelength band. However, as a key component, the performance of current high-speed photodetectors at the 2 μm wavelength is still not comparable with those at the 1.55 μm wavelength band, which chokes the feasibility of the new spectral window. In this work, we demonstrate, for the first time to our knowledge, a high-speed uni-traveling carrier photodiode for 2 μm applications with InGaAs/GaAsSb type-II multiple quantum wells as the absorption region, which is lattice-matched to InP. The devices have the responsivity of 0.07 A/W at 2 μm wavelength, and the device with a 10 μm diameter shows a 3 dB bandwidth of 25 GHz at − 3 V bias voltage. To the best of our knowledge, this device is the fastest photodiode among all group III-V and group IV photodetectors working in the 2 μm wavelength range.
© 2019 Optical Society of America under the terms of the OSA Open Access Publishing Agreement
1. INTRODUCTION
Due to the exponentially increasing volume of internet traffic, today’s optical communication systems are rapidly approaching their capacity limit [ 1 , 2 ]. This “capacity crunch” provides an impetus for developing next-generation optical communication systems. A new spectral window at the 2 μm wavelength is a promising solution to increase the system capacity due to the availability of low-loss (0.2 dB/km) hollow-core photonic bandgap fiber (HC-PBGF) and the high-bandwidth (from 1810 to 2050 nm) thulium-doped fiber amplifier (TDFA) at 2 μm wavelength [ 3 , 4 ]. Studies on 2 μm optical components and system architecture have shown the promising potential of this new spectral window [ 5 – 7 ]. An eight-channel wavelength-division multiplexing transmission system with 100 Gbit/s data capacity has been demonstrated at the 2 μm band [ 6 ].
A high-speed photodetector is one of the key components of the optical communication system, and among various figures of merit, bandwidth is most commonly used for a high-speed-device benchmark. At the 2 μm wavelength band, high-speed photodiodes have been demonstrated with In-rich InGaAs on InP [ 5 , 7 – 11 ], InGaAsSb on GaSb [ 12 ], GeSn/Ge on Si [ 13 , 14 ], and defect-mediated Si [ 15 ]. In 0.53 Ga 0.47 As on InP, which cuts off at 1.7 μm, is the most widely used absorption material for high-speed application due to its high carrier mobility. For 2 μm operation, In-rich InGaAs has to be used to extend thecutoff wavelength. Ye et al. demonstrated a 10 GHz bandwidth photodiode with In 0.7 Ga 0.3 As as an absorption layer [ 11 ]. Joshi et al. achieved a 16 GHz bandwidth using a In 0.72 Ga 0.28 As absorber [ 9 ]. A partially depleted photodiode with a Ga 0.8 In 0.2 As 0.16 Sb 0.84 absorber on GaSb has achieved a 3 dB bandwidth of 6 GHz [ 12 ]. On the other hand, a GeSn/Ge quantum well grown on Si shows a benefit for integration with other silicon devices, and a Ge 0.92 Sn 0.08 / Ge photodiode with a 10 GHz bandwidth has been reported [ 14 ]. By inserting lattice defects, a silicon-on-insulator photodiode can also operate at the 2 μm wavelength, and a 15 GHz bandwidth can be achieved [ 15 ]. However, the performance of these devices is sensitive to the crystal quality, making them inconvenient for practical application.
Short-wavelength infrared (SWIR) photodetectors using InGaAs/GaAsSb type-II multiple quantum wells (MQWs) on the InP substrate as an absorber have been demonstrated and well-studied recently [ 16 – 19 ]. The devices enjoy the advantage of lattice-matched property on InP, and show low dark current and high detectivity at room temperature. The crystal quality of the MQW structure can be ensured since it is lattice-matched to InP and the growth technique of InP material system is mature. A PIN-based high-speed photodiode with InGaAs/GaAsSb MQWs for 2 μm operation has achieved a 3 dB bandwidth in the range of 3.5 GHz to 10 GHz, which is mainly limited by the slow transport of an optical-generated hole in the absorption region [ 20 – 22 ].
To further improve the bandwidth, a uni-traveling carrier photodiode (UTC-PD) with a type-II MQW absorber was proposed and theoretically investigated in our previous work [ 23 ]. A UTC-PD based on a InGaAs/InP material system has been demonstrated with hundreds of gigahertz bandwidth at a 1.55 μm wavelength band for high-speed application [ 24 – 27 ]. In the UTC-PD structure, the optical-generated carriers are excited in the undepleted p-type absorption layer, and only electrons are injected into the InP drift layers; thus, the effective carrier transit time is shorter than that of the PIN structure with the proper design.
In this work, we demonstrate the normal-incident InGaAs/GaAsSb MQW UTC-PDs at the 2 μm wavelength band with a 3 dB bandwidth of 25 GHz and bit rate of 30 Gbit/s. To the best of our knowledge, this is the highest bandwidth and bit rate demonstrated at the 2 μm wavelength band. By analyzing the RF performance of the devices with different diameters, it is found that the 3 dB bandwidth performance can be further improved by optimizing the fabrication process.
2. DEVICE STRUCTURE AND FABRICATION
The epilayer structure of the photodiode is shown in Fig. 1(a) . All layers are lattice-matched to the InP substrate. The structure was grown on semi-insulating double-side-polished InP substrate by a molecular beam epitaxy (MBE) system. The epitaxial growth began with a 200 nm n + InP layer, 20 nm n + InGaAs layer, and 900 nm n + InP layer. The 100 nm n-doped InP layer with a doping concentration of 1 × 10 18 cm − 3 was then grown to reduce Si diffusion into the 400 nm intrinsic InP drift layer. The absorption layer consists of 180 nm graded doped 3 nm/3 nm In 0.53 Ga 0.47 As / GaAs 0.50 Sb 0.50 MQWs, which is expected to have fast response speed while maintaining a cutoff wavelength larger than 2 μm, according to our simulation [ 23 ]. Following the MQW layers is the 30 nm p-doped large bandgap AlGaAsSb electron blocking layer to prevent the diffusing of electrons in the absorption layer towards the p-doped InGaAs contact layer on the top. Room-temperature photoluminescence (PL) of the epitaxial layers was conducted by using the 532 nm wavelength laser as the excitation source and a Fourier transform infrared (FTIR) spectrometer with an In-rich InGaAs detector. Two peaks can be identified in Fig. 1(b) , indicating the good crystal quality of the material. The peak at 2.1 μm corresponds to the transition between the ground states in InGaAs and GaAsSb quantum well layers, respectively, and the second peak at 1.75 μm may correspond to the emission from the electron ground state to light hole state, as shown in Fig. 1(d) .
Fig. 1. (a) Epitaxial structure of the type-II MQW UTC photodiode. (b) Photoluminescence measurement result of the epitaxial structure. (c) Schematic diagram of the fabricated device. (d) Band diagram of the MQW absorber. The wave functions and potential transitions are shown. The wave functions are calculated by a kp method, and the two transitions correspond to the peaks at 2.1 μm and 1.75 μm in the photoluminescence spectrum.
Download Full Size | PDF
After the material growth, the epitaxial structure was processed into double-mesa structures, as shown in Fig. 1(c) . A set of mesa devices with different diameters was formed by standard photolithography and a wet-etching process. A 150 μm pitch ground–signal–ground (GSG) coplanar waveguide (CPW) pad with an air-bridge structure was electroplated for high-frequency measurement. The substrate is backside-polished to support backside illumination with no antireflection coating.
3. MEASUREMENT RESULT
A. electrical characteristics.
The dark current voltage (I-V) curves of the photodiodes at room temperature are shown in Fig. 2 . The dark current value at − 3 V is 3.02 nA, 3.88 nA, and 5.82 nA for the photodiodes with diameters of 10 μm, 20 μm, and 40 μm, respectively, which are lower than the In-rich InGaAs photodiodes [ 5 , 7 – 11 ]. Figure 3 shows the capacitance-voltage (C-V) curves. The device is fully depleted at − 1 V , and the capacitance at − 3 V is 60.6 fF, 154.0 fF, and 432.6 fF for the 10 μm, 20 μm, and 40 μm diameter photodiodes, respectively. Parasitic capacitance of 49.6 fF was found based on the linear fitting of capacitance versus device area, as shown in Fig. 4 , which indicates that optimization is needed for future fabrication processes. The origin of the parasitic capacitance is explained in detail in Supplement 1 .
Fig. 2. Dark current characteristics for three devices with different diameters at room temperature.
Fig. 3. Measured capacitance versus reverse bias for three devices with different diameters at room temperature.
Fig. 4. Capacitance of devices at − 3 V bias. The fitting result indicates a parasitic capacitance of 49.6 fF.
B. Responsivity
The top-illuminated responsivity spectrum was measured using an FTIR spectrometer. A standard blackbody radiation source at 700°C was used to calibrate the responsivity. As shown in Fig. 5 , the 100% cutoff wavelength is around 2.25 μm, and the responsivity is 0.07 A/W at 2 μm wavelength and reverse bias (larger than − 1 V ). Considering the 180 nm thickness of the absorption layer, the equivalent absorption coefficient of the absorption layer is about 3600 cm − 1 , which is higher than the simulation value of 2000 cm − 1 in [ 22 ].
Fig. 5. Responsivity spectrum at various bias voltages. The inset shows the responsivity at 2 μm wavelength.
C. Bandwidth
The 3 dB bandwidth was measured by a heterodyne setup with two beams of laser light at a wavelength of 2004 nm coupled together, which generates an intensity-modulated light with a frequency from hundreds of megahertz to more than 30 GHz. The modulated light was amplified, and then it illuminated the devices from backside. The details of the measurement setup are shown in Fig S2 of Supplement 1 .
Figure 6 shows the frequency response of a 10 μm diameter photodiode at different bias voltages. The 3 dB bandwidth increases slightly while reverse bias increases. The highest 3 dB bandwidth of 25 GHz can be achieved at − 4 V bias. Theoretically, the 3 dB bandwidth of the photodiode is often limited by transit time and RC time, as expressed by the equation (1) f 3 dB = ( 1 f T 2 + 1 f RC 2 ) − 1 , where f 3 dB is the total 3 dB bandwidth, f T is the transit time limit bandwidth, and f RC is the RC limit bandwidth. Recall that in the C-V measurement results shown in Fig. 4 , the junction capacitance is almost constant once fully depleted (larger than − 1 V reverse bias). As a result, the small increase in the 3 dB bandwidth should be caused by the enhancement in the transit process. When large bias is applied, the depletion region will extend to the absorption region and accelerate the carrier transport in the absorption region. The bandwidth might be further improved by increasing the bias voltage, but that may cause a reliability issue and permanent breakdown failure of the device.
Fig. 6. Frequency response of a 10 μm photodiode at various bias voltages. The photocurrent is set to 1 mA. The inset shows the 3 dB bandwidth at different bias voltages.
Figure 7 shows the frequency response of the 10 μm photodiode at different photocurrents. The 3 dB bandwidth is almost the same (around 25 GHz at − 3 V ) when the photocurrent increases from 1 mA to 10 mA, and no obvious saturation can be found.
Fig. 7. Frequency response of a 10 μm photodiode at different photocurrents. The bias voltage is − 3 V .
Figure 8 shows the frequency response of three photodiodes with different diameters. The 3 dB bandwidths of the 40 μm, 20 μm, and 10 μm devices are 5 GHz, 15 GHz, and 25 GHz, respectively. The smaller photodiode has smaller junction capacitance, leading to a larger RC limit bandwidth, while the transit limit bandwidth remains the same. Thus, the strong bandwidth dependency on diameter indicates that the 3 dB bandwidth of the devices is dominated by the RC limit. To verify the RC limit, we measured the S-parameters and fitted the parameters with an equivalent circuit model, as shown in Fig. 9(a) . The fitting curves are shown in Figs. 9(b) , 9(c) , and 9(d) . The extracted model parameters are listed in Table 1 . Then, the theoretical RC limit frequency response can be calculated using the equivalent model, as shown in Fig. 9(e) . The RC limit bandwidths of the 40 μm, 20 μm, and 10 μm devices are 7.7 GHz, 18.9 GHz, and 41.4 GHz, respectively. Applying Eq. ( 1 ), a rough estimation of the transit time limit bandwidth for the 10 μm diameter photodiode is about 31 GHz. As shown in Fig. 4 , a parasitic capacitance of 49.6 fF exists in our devices, and so the 3 dB bandwidth of the devices can be further improved by reducing the parasitic capacitance. Figure 10 reviews the 3 dB bandwidth of high-speed photodetectors operating at the 2 μm wavelength in recent years. With proper design, the InGaAs/GaAsSb MQWs UTC-PD can achieve better bandwidth performance than other devices.
Fig. 8. Frequency response of photodiodes with different diameters. The bias voltage is − 3 V , and the photocurrent is 1 mA.
Fig. 9. (a) Equivalent circuit model used in parameter fitting. C j is the junction capacitance, R s is the series resistance (resistance of the ohm contacts and the CPW pads), L s is the inductance of the CPW pads, and R j is the junction resistance, which is hundreds of megaohms and can be regarded as open circuit. The measured and fitting curves of S11 of (b) 10 μm, (c) 20 μm, and (d) 40 μm photodiodes at − 3 V bias (the blue curve is the measured data while the red curve is the fitting curve). (e) Calculated RC limit frequency response using the fitting results.

Table 1. Fitting Parameters
Fig. 10. Review of the 3 dB bandwidth of high-speed photodiodes operating at 2 μm wavelength reported in recent years.
Figure 11 shows the eye diagram of a 10 μm photodiode biased at − 3 V and operating at a 20 Gbit/s, 25 Gbit/s, and 30 Gbit/s data rate. The 128 bits 2^15–1 pseudo-random binary sequence (PRBS) sequences were generated as the data source to drive a 2 μm wavelength Mach–Zehnder modulator, which modulates the optical signal coming from a 2004 nm wavelength single-frequency fiber laser with a modulation depth of 0.25. The optical power illuminating the photodiode is about 7 mW. The output of the photodiode was amplified by a + 23 dB microwave amplifier and then displayed on the real-time sampling oscilloscope. A clear eye pattern is demonstrated at a 30 Gbit/s data rate, which indicates the devices can be used at a 2 μm optical communications system.
Fig. 11. Eye pattern of a 10 μm photodiode at 20 Gbit/s, 25 Gbit/s, and 30 Gbit/s.
D. Saturation Power
For some applications, such as microwave photonics, the high-speed photodetector works in high-power mode, and the saturation power is another important figure of merit. When the photocurrent is high, a large number of electrons exists in the depletion region, and the electrical field in the depletion region might collapse due to the space charge effect. This limits the carrier sweeping out process, causing a saturation in output power. Figures 12 and 13 show the relationship between the output power and the photocurrent. In this measurement, lasers of 1.55 μm wavelength were used as the optical source due to the power limitation of the 2 μm optical amplifier. Notice that the saturation characteristics should be the same for 1.55 μm and 2 μm input, since the carrier transports in the depletion region are identical. The ideal output power of a photodetector can be calculated by (2) P ideal = m 1 2 I p 2 R L , where I p is the photocurrent, R L is the load resistance, and m is the modulation depth, which is almost 1 in our measurement. The compression in the figures is the difference between the actual output power and the ideal output power. The 1 dB compression point is defined as the point that the compression drops 1 dB compared to its maximal value, and it describes the power handling ability of the devices. Table 2 lists the 1 dB compression point of 10 μm and 20 μm devices at different bias voltage. The saturation power increases with the increase of the bias voltage, since the stronger electrical field can endure more space charge effect. One way to further improve the saturation power is to use charge-compensated doping in the depletion region [ 28 ]. With proper design, the ionized dopant charges will neutralize the charges of the optical-generated carriers, thus avoiding the collapse of the electrical field and making the device suitable for high-power operation.
Fig. 12. Output RF power and compression versus photocurrent for a 10 μm photodiode at 25 GHz and at different bias voltages. The gray dashed line shows the ideal output power.
Fig. 13. Output RF power and compression versus photocurrent for a 20 μm photodiode at 15 GHz and at different bias voltages. The gray dashed line shows the ideal output power.
Table 2. 1 dB Compression Points
4. CONCLUSION
In this work, we have reported a high-speed photodiode working at the 2 μm wavelength based on InGaAs/GaAsSb type-II MQWs with uni-traveling carrier design. The InGaAs/GaAsSb type-II MQW absorber cuts off at 2.25 μm, and the responsivity is 0.07 A/W at 2 μm. The 3 dB bandwidths at 2 μm are 25 GHz, 15 GHz, and 5 GHz for photodiodes with 10 μm, 20 μm, and 40 μm diameter, respectively. A clear eye pattern can be observed at 30 Gb/s for the 10 μm photodiode. Analysis shows that the bandwidth of the current device can still be improved with further reduction of the parasitic capacitance by optimizing the fabrication process. The excellent high-speed performance of this device paves the way towards more promising feasibility of a new spectral window at the 2 μm wavelength.
Shanghai Sailing Program (17YF1429300); ShanghaiTech University startup funding (F-0203-16-002).
See Supplement 1 for supporting content.
1. A. D. Ellis, J. Zhao, and D. Cotter, “Approaching the non-linear Shannon limit,” J. Lightwave Technol. 28 , 423–433 (2010). [ CrossRef ]
2. D. J. Richardson, “Filling the light pipe,” Science 330 , 327–328 (2010). [ CrossRef ]
3. P. J. Roberts, F. Couny, H. Sabert, B. J. Mangan, D. P. Williams, L. Farr, M. W. Mason, A. Tomlinson, T. A. Birks, J. C. Knight, and P. St.J. Russell, “Ultimate low loss of hollow-core photonic crystal fibres,” Opt. Express 13 , 236–244 (2005). [ CrossRef ]
4. Z. Li, A. M. Heidt, N. Simakov, Y. Jung, J. M. O. Daniel, S. U. Alam, and D. J. Richardson, “Diode-pumped wideband thulium-doped fiber amplifiers for optical communications in the 1800–2050 nm window,” Opt. Express 21 , 26450–26455 (2013). [ CrossRef ]
5. B. Corbett, M. R. Gleeson, N. Ye, C. Robert, H. Yang, H. Zhang, N. M. Suibhne, and F. C. G. Gunning, “InP-based active and passive components for communication systems at 2 μm,” J. Lightwave Technol. 33 , 971–975 (2014). [ CrossRef ]
6. H. Zhang, N. Kavanagh, Z. Li, J. Zhao, N. Ye, Y. Chen, N. V. Wheeler, J. P. Wooler, J. R. Hayes, S. R. Sandoghchi, F. Poletti, M. N. Petrovich, S. U. Alam, R. Phelan, J. O’Carroll, B. Kelly, L. Grüner-Nielsen, D. J. Richardson, B. Corbett, and F. C. Garcia Gunning, “100 Gbit/s WDM transmission at 2 μm: transmission studies in both low-loss hollow core photonic bandgap fiber and solid core fiber,” Opt. Express 23 , 4946–4951 (2015). [ CrossRef ]
7. F. C. Garcia Gunning, N. Kavanagh, E. Russell, R. Sheehan, J. O’Callaghan, and B. Corbett, “Key enabling technologies for optical communications at 2000 nm,” Appl. Opt. 57 , E64–E70 (2018). [ CrossRef ]
8. A. Joshi and D. Becker, “High-speed low-noise p-i-n InGaAs photoreceiver at 2-μm wavelength,” IEEE Photon. Technol. Lett. 20 , 551–553 (2008). [ CrossRef ]
9. A. Joshi and S. Datta, “High-speed, large-area, p-i-n InGaAs photodiode linear array at 2-micron wavelength,” Proc. SPIE 8353 , 83533D (2012). [ CrossRef ]
10. H. Yang, B. Kelly, W. Han, F. Gunning, B. Corbett, R. Phelan, J. O’Carroll, H. Yang, F. H. Peters, X. Wang, N. Nudds, P. O’Brien, N. Ye, and N. MacSuibhne, “Butterfly packaged high-speed and low leakage InGaAs quantum well photodiode for 2000nm wavelength systems,” Electron. Lett. 49 , 281–282 (2013). [ CrossRef ]
11. N. Ye, H. Yang, M. Gleeson, N. Pavarelli, H. Y. Zhang, J. O’Callaghan, W. Han, N. Nudds, S. Collins, A. Gocalinska, E. Pelucchi, P. O’Brien, F. C. G. Gunning, F. H. Peters, B. Corbett, J. O. Callaghan, W. Han, N. Nudds, S. Collins, E. Pelucchi, P. O. Brien, F. C. G. Gunning, F. H. Peters, and B. Corbett, “InGaAs surface normal photodiode for 2 μm optical communication systems,” IEEE Photon. Technol. Lett. 4 , 1469–1472 (2015). [ CrossRef ]
12. J. M. Wun, Y. W. Wang, Y. H. Chen, J. E. Bowers, and J. W. Shi, “GaSb-based p-i-n photodiodes with partially depleted absorbers for high-speed and high-power performance at 2.5-μm wavelength,” IEEE Trans. Electron Devices 63 , 2796–2801 (2016). [ CrossRef ]
13. Y. Dong, W. Wang, S. Xu, D. Lei, X. Gong, X. Guo, H. Wang, S.-Y. Lee, W.-K. Loke, S.-F. Yoon, and Y.-C. Yeo, “Two-micron-wavelength germanium-tin photodiodes with low dark current and gigahertz bandwidth,” Opt. Express 25 , 15818–15827 (2017). [ CrossRef ]
14. S. Xu, W. Wang, Y.-C. Huang, Y. Dong, S. Masudy-Panah, H. Wang, X. Gong, and Y.-C. Yeo, “High-speed photo detection at two-micron-wavelength: technology enablement by GeSn/Ge multiple-quantum-well photodiode on 300 mm Si substrate,” Opt. Express 27 , 5798–5813 (2019). [ CrossRef ]
15. J. J. Ackert, D. J. Thomson, L. Shen, A. C. Peacock, P. E. Jessop, G. T. Reed, G. Z. Mashanovich, and A. P. Knights, “High-speed detection at two micrometres with monolithic silicon photodiodes,” Nat. Photonics 9 , 393–396 (2015). [ CrossRef ]
16. B. Chen, “SWIR/MWIR InP-based p-i-n photodiodes with InGaAs/GaAsSb type-II quantum wells,” IEEE J. Quantum Electron. 30 , 399–402 (2011).
17. B. Chen, W. Y. Jiang, J. Yuan, A. L. Holmes, and B. M. Onat, “Demonstration of a room-temperature InP-based photodetector operating beyond 3 μm,” IEEE Photon. Technol. Lett. 23 , 218–220 (2011). [ CrossRef ]
18. B. Chen and A. L. Holmes, “InP-based short-wave infrared and midwave infrared photodiodes using a novel type-II strain-compensated quantum well absorption region,” Opt. Lett. 38 , 2750–2753 (2013). [ CrossRef ]
19. B. Chen, W. Y. Jiang, and A. L. Holmes Jr., “Design of strain compensated InGaAs/GaAsSb type-II quantum well structures for mid-infrared photodiodes,” Opt. Quantum Electron. 44 , 103–109 (2012). [ CrossRef ]
20. B. Tossoun, R. Stephens, Y. Wang, S. Addamane, G. Balakrishnan, A. Holmes, and A. Beling, “High-speed InP-based p-i-n photodiodes with InGaAs/GaAsSb type-II quantum wells,” IEEE Photon. Technol. Lett. 30 , 399–402 (2018). [ CrossRef ]
21. Y. Chen, X. Zhao, J. Huang, Z. Deng, C. Cao, Q. Gong, and B. Chen, “Dynamic model and bandwidth characterization of InGaAs/GaAsSb type-II quantum wells PIN photodiodes,” Opt. Express 26 , 35034–35045 (2018). [ CrossRef ]
22. B. Tossoun, J. Zang, S. J. Addamane, G. Balakrishnan, A. L. Holmes, and A. Beling, “InP-based waveguide-integrated photodiodes with InGaAs/GaAsSb type-II quantum wells and 10-GHz bandwidth at 2 μm wavelength,” J. Lightwave Technol. 36 , 4981–4987 (2018). [ CrossRef ]
23. Y. Chen and B. Chen, “Design of InP-based high-speed photodiode for 2-μm wavelength application,” IEEE J. Quantum Electron. 55 , 1–8 (2019). [ CrossRef ]
24. J. M. Wun, Y. W. Wang, and J. W. Shi, “Ultrafast uni-traveling carrier photodiodes with GaAs 0.5 Sb 0.5 /In 0.53 Ga 0.47 As type-II hybrid absorbers for high-power operation at THz frequencies,” IEEE J. Sel. Top. Quantum Electron. 24 , 1–7 (2018). [ CrossRef ]
25. C. C. Renaud, M. Natrella, C. Graham, J. Seddon, F. Van Dijk, and A. J. Seeds, “Antenna integrated THz uni-traveling carrier photodiodes,” IEEE J. Sel. Top. Quantum Electron. 24 , 1–11 (2018). [ CrossRef ]
26. A. Beling, X. Xie, and J. C. Campbell, “High-power, high-linearity photodiodes,” Optica 3 , 328–338 (2016). [ CrossRef ]
27. T. Ishibashi, Y. Muramoto, T. Yoshimatsu, and H. Ito, “Unitraveling-carrier photodiodes for terahertz applications,” IEEE J. Sel. Top. Quantum Electron. 20 , 79–88 (2014). [ CrossRef ]
28. N. Li, X. Li, S. Demiguel, X. Zheng, J. C. Campbell, D. A. Tulchins, K. J. Williams, T. D. Isshiki, G. S. Kinsey, and R. Sudharsansan, “High-saturation-current charge-compensated InGaAs-InP uni-traveling-carrier photodiode,” IEEE Photon. Technol. Lett. 16 , 864–866 (2004). [ CrossRef ]
Supplementary Material (1)
Optica participates in Crossref's Cited-By Linking service . Citing articles from Optica Publishing Group journals and other participating publishers are listed here.
Alert me when this article is cited.
Figures (13)
Equations (2)
Equations on this page are rendered with MathJax. Learn more.
Prem Kumar, Editor-in-Chief
Fitting Parameters
1 dB Compression Points
Confirm Citation Alert
Field error.
- Publishing Home
- Conferences
- Preprints (Optica Open)
- Information for
- Open Access Information
- Open Access Statement and Policy
- Terms for Journal Article Reuse
- About Optica Publishing Group
- About My Account
- Send Us Feedback
- Optica Home
- Other Resources
- Optica Open
- Optica Publishing Group Bookshelf
- Optics ImageBank
- Optics & Photonics News
- Spotlight on Optics
- Go to My Account
- Login to access favorites
- Recent Pages
Login or Create Account
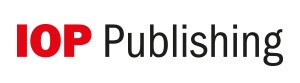
We apologize for the inconvenience...
To ensure we keep this website safe, please can you confirm you are a human by ticking the box below.
If you are unable to complete the above request please contact us using the below link, providing a screenshot of your experience.
https://ioppublishing.org/contacts/

IMAGES
VIDEO
COMMENTS
Uni-traveling-carrier photodiodes Abstract: A photodiode (PD) that can provide both high speed and high saturation output is of great importance for the development of future large-capacity fiber-optic communication systems and ultrafast measurement systems. A high output PD combined with an erbium-doped fiber amplifier can eliminate the need ...
The uni-traveling-carrier photodiode (UTC-PD) is a kind of pin junction photodiode that selectively uses electrons as active carriers. The diode structure has a relatively thin p-type absorber where electrons are generated as minority carriers, and then they diffuse and/or field-accelerate toward the collector. Since the electrons travel in the ...
Monolithic III-V photodiodes 5,6 ——often uni-traveling-carrier photodiodes (UTC PDs)—also show very high bandwidths, but these lossy waveguide platforms are not suitable for large scale photonic integration. On the other hand, the current solutions to bring III-V photodiodes to SiN show only limited bandwidths.
Si/Ge uni-traveling carrier photodiodes exhibit higher output current when space-charge effect is overcome and the thermal effects is suppressed. High current is beneficial for increasing the ...
Uni-traveling-carrier photodiode (UTC-PD) is a newly developed high-speed photodiode for generating high output current. The high saturation current is realized by its operation mode in which only ...
A new ultrafast photodiode, uni-traveling-carrier photodiode (UTC-PD) is proposed, and its photoresponse characterization on fabricated devices is presented. The prime feature of this PD is much higher output saturation current than that in a conventional pin-PD. This is achieved by reducing the space charge effect by utilizing electron velocity overshoot in the carrier collecting layer. A 20 ...
We demonstrate the capability of fabricating extremely high-bandwidth Uni-Traveling Carrier Photodiodes (UTC-PDs) using techniques that are suitable for active-passive monolithic integration with Multiple Quantum Well (MQW)-based photonic devices. The devices achieved a responsivity of 0.27 A/W, a 3-dB bandwidth of 170 GHz, and an output power of −9 dBm at 200 GHz. We anticipate that this ...
In this article, we report an uni-traveling-carrier photodiode (UTC-PD) incorporating nanoscale optical microstructures. We have designed a UTC-PD incorporating a core-cladding confinement structure, which incorporates internal optical scattering structures to convert incident light from vertical incidence to lateral propagation, and to as much as possible confine light within the absorption ...
A modified uni-traveling-carrier photodiode (MUTC-PD) with an optimized depletion region is fabricated and its saturation characteristics are investigated. The space-charge effect is effectively suppressed by inserting a carefully designed cliff layer in the middle of the depletion region. The 22-µm-diameter device exhibits a bandwidth of 28 GHz.
InP-InGaAs uni-traveling-carrier photodiode with improved 3-dB bandwidth of over 150 GHz. Uni-traveling-carrier photodiodes (UTC-PD's) with ultrafast response and high-saturation output are reported. It is experimentally demonstrated that the photoresponse of UTC-PD's is improved by….
In this work, we have reported a high-speed photodiode working at the 2 μm wavelength based on InGaAs/GaAsSb type-II MQWs with uni-traveling carrier design. The InGaAs/GaAsSb type-II MQW absorber cuts off at 2.25 μm, and the responsivity is 0.07 A/W at 2 μm. The 3 dB bandwidths at 2 μm are 25 GHz, 15 GHz, and 5 GHz for photodiodes with 10 ...
The development of uni-traveling carrier photodiodes over the past 25 years is briefly reviewed and the key design in their structure are discussed. Monte Carlo simulation run for minority electrons locally excited in a p-type InGaAs structure reveals how they behave and leave the absorber. This shows the very diffusive nature of electrons, even in a moderately high quasi-field.
Unlike conventional p-i-n photodiode, the uni-traveling carrier photodiode (UTC-PD) consists of a p-type neutral absorption layer and a wide-gap depleted collection layer [1, 2]. This means that the thickness of the depleted collector can be designed to be independent of the thickness of the absorber. Therefore, the RC delay time is decreased ...
1Bauman Moscow State Technical University, Moscow 105005, Russia ... :10 Hz from the carrier optical frequency, whereas precise noise measurement in this range is challenging [10]. ... signal through WDM goes to photodiodes with sensitivity at 1550 nm equal to 0.9 A/W, so we get
range both Standing(SW) and Travelling Wave (TW) oper-ations are possible. For comparison, rf parameters for TW Disk Loaded Waveguide (DLW) as well as for SW Coupled Cells Structures (CCS) have been optimized at an operat-ing frequency of 1300 MHz in a wide range of cells di-mensions. General properties for the two operating modes
Prof. Gregory N. Goltsman. Gregory Goltsman received his PhD degree in radio-physics and ScD degree in physics of semiconductors and dielectrics from Moscow State Pedagogical University (MSPU), Moscow, Russia, in 1973 and 1985, respectively. Currently, he is Professor and Chairman of General and Experimental Physics Department, and also the ...
Energies 2022, 15, 9193 4 of 8 350 to 1600 C at a rate of about 8.0 C/min had visible cracks.For this reason, the two modes shown in Figure2with heating rates of no more than 4.0-5.0 C/min were chosen as working modes. 1XVXXð1W[ð1_W_Y1 Z1 1 _1